ABSTRACT
Heterotrophic microalgae, capable of converting organic carbons to biofuel, as well as assimilating nutrients, have a great prospective in wastewater treatment. Meanwhile, the knowledge about heterotrophic microalgae is still far less than the autotrophic one. Hence, in this study, 20 heterotrophic microalgal strains were isolated from a domestic wastewater treatment plant, and identified based on morphology and partial 18S and 23S rRNA gene sequences. Further, their biological traits and applicability in real wastewater treatment were assessed in terms of N, P, TOC removal efficiency, growth parameters, self-sellteability and lipids production.
The results showed that four selected strains (i.e.,
Botryococcus sp. NJD-1,
Scenedesmus sp. NJD-2,
Scenedesmus sp. NJD-5 and
Chlorella sp. NJD-3) performed well in untreated domestic wastewater, achieving the maximum biomass yield (g L
-1) of 1.2 ± 0.002, 2.0 ± 0.03, 1.6 ± 0.01 and 0.54 ± 0.03, within the highest lipid content (g g
-1 DCW) of 61.7%, 55.9%, 50.4% and 40.1%, respectively. Among 11 isolates tested for the tendency to settle,
Chlorella sp. NJD-8 showed the highest settleability efficiency of 54.2% after 30 min.
Botryococcus sp. NJD-1 exposed a potential capacity to assimilate a wide range of carbon substrates. Comprehensively, three strains were recommended for real wastewater treatment, i.e.,
Botryococcus sp. NJD-1 with up to 64.5% of N-NO
3 removal,
Scenedesmus sp. NJD-2 by 96.1% of P-PO
4 removal, and
Scenedesmus sp. NJD-5 with 99.9% of TOC removal.
Keywords: Microalgae; Isolation; Wastewater treatment; Heterotrophic cultivation; Biofuel.
ABBREVIATIONS
µ Specific growth rate
ACVI Algal culture volume index
ADE Autoclaved dairy effluent
AMWW Autoclaved municipal wastewater
APHA American public health association
AR Analytical reagent
ARDWW Autoclaved real domestic wastewater
ATP Adenosine Triphosphate
BLAST Basic local alignment search tool
BOD Biochemical oxygen demand
CMWW Concentrated municipal wastewater
CO2 Carbon dioxide
COD Chemical oxygen demand
DCW Dry cell weight
DE Dairy effluent
DNA Deoxyribo nucleic acid
DT Doubling time
DWWTP Domestic wastewater treatment plant
FAMES Fatty acid methyl esters
kWh Kilowatt-hour
LC Lipid content
MBG11 Modified blue green media
MBold-3N Modified Bold-3N media
MWW Municipal wastewater treatment
NADPH Nicotinamide adenine dinucleotide phosphate
NCBI National center for biotechnology information
OD Optical density
PBR Photobioreactor
PCR Polymerase chain reaction
RDWW Real domestic wastewater
RE Removal efficiency
RNA Ribonucleic acid
rpm Revolutions per minute
RR Removal rate
rRNA Ribosomal ribonucleic acid
SE Settling efficiency
sp. Species
SV Settled volume
SVI Sludge volume index
TN Total nitrogen
TOC Total organic carbon
TSS Total suspended solid
UCMWW Autoclaved concentrated municipal wastewater
URDWW Untreated real domestic wastewater
UV/Vis Ultraviolet–visible
WWTP Wastewater treatment plant
Chapter 1 Literature Review
1.1 Overview of wastewater treatment
1.1.1 Wastewaters
Wastewater is defined as any water that has been adversely affected in quality by anthropogenic activities. Wastewater can originate from a combination of domestic, industrial, commercial or agricultural actions, surface runoff or storm water, and from sewer inflow or infiltration [
1]. {Tilley, 2008 #721;Liu, 2014 #825}Wastewaters provide not only water medium but also most of the necessary nutrients suitable for cultivation of microalgae evidenced by high algae growth rate and productivity and nutrient removal efficiency. Therefore, coupling wastewater treatment with algae cultivation may offer an economically viable and environmentally friendly way for sustainable renewable algae-based biofuel and bio-based chemicals production as well as credits for wastewater treatment [
2]. Municipal wastewater or sewage is usually carried out in a combined sanitary sewer and treated at a wastewater treatment plant. Via an effluent pipe, a treated wastewater is discharged into receiving water. Wastewaters generated in areas without access to centralized sewer systems rely on on-site wastewater systems. These typically comprise a septic tank, drain field, and optionally an on-site treatment unit. The management of wastewater belongs to the overarching term sanitation, just like the management of human excreta, solid waste and storm water (drainage). Sewage is a type of wastewater that comprises domestic wastewater and is therefore contaminated with feces or urine from people's toilets, but the term sewage is also used to mean any type of wastewater. Sewerage as the physical infrastructure, including pipes, pumps and screens, channels etc. used to deliver sewage from its origin to the point of eventual treatment or disposal.
1.1.2 Wastewater treatment
Wastewater treatment is the process of removing pollutants from wastewater through serial physical, chemical and biological processes. Its purpose is to produce an environmentally safe treated effluent and a solid waste suitable for disposing or re-using [
3]. However, there are numerous processes that can be used to clean up wastewaters depending on the type and extent of contamination. Likewise, wastewater can be treated in wastewater treatment plants through several ways including physical, chemical and biological treatment processes. Municipal wastewater treatment (MWW) is treated in sewage treatment plants, which may also be referred to as wastewater treatment plants. Also agricultural wastewater may be treated in agricultural wastewater treatment processes, whereas industrial wastewater can also be treated in industrial wastewater treatment processes.
To deal with the negative effects of wastewater on the recipient, traditional wastewater treatment generally involves three stages [
4]. The first stage is the primary treatment in which wastewater is held in a motionless basin, where heavy solids and colloids can settle to the bottom, while light solids and colloids can float to the top. It targets at removing large particles in the sewage by means of grids or sedimentation. The settled and floating materials are removed and the remaining liquid is discharged to secondary stage. In the secondary wastewater treatment, microorganisms are used to remove dissolved and suspended matter in the wastewater in a managed habitat and then the microorganisms are separated from the liquid. It aims as reducing the biochemical oxygen demand (BOD) in the wastewater by oxidizing organic compounds and ammonium. This process is often carried out in aerated tanks called activated sludge and it involves both heterotrophic bacteria and protozoa. The bacteria degrade the organic material and the protozoa graze the bacteria, and in both cases organic material is converted to CO
2 and water [
4]. Additionally, primary treatment removes about 60 percent of suspended solids from wastewater and also involves aerating the wastewater, to put oxygen back in whereas secondary treatment removes more than 90 percent of suspended solids (https://water.usgs.gov/edu/wuww.html).
The next treatment process after secondary treatment is the tertiary wastewater treatment, in which more advanced techniques such as carbon adsorption; flocculation, precipitation, etc. are used to remove the remaining stubborn contaminants in the wastewater to raise the effluent quality before it is discharged to the receiving environment [
4]. It mainly aims at removing nitrogen (N) and phosphorus (P) nutrients. N may be removed by nitrification-denitrification processes, where the ammonium is first oxidized to nitrate by nitrifying bacteria in aerobic reactors, and thereafter recycled to an oxygen free reactor where it is converted to nitrogen gas (N
2) by denitrifying bacteria. The first step in the nitrification is oxidation of ammonium to nitrite (1.1) and the second step is the oxidation of nitrite to nitrate (1.2):
2NH4+ + 3O2 → 2NO2‐ + 4H+ + 2H2O (1.1)
2NO2‐ + O2 → 2NO3‐ (1.2)
Autotrophic bacteria belonging to the family
Nitrobacteriaceae perform the nitrification. On the other hand, denitrification is carried out by a large number of heterotrophic bacteria, which all need organic carbon for growth. The denitrification reaction, performed according to the following reaction, has also nitrite as an intermediary step:
NO3‐ → NO2‐ → NO → N2O → N2 (1.3)
Phosphorus in wastewater is most often removed by chemical precipitation with aluminium or iron salts to form aluminium or ferric phosphate:
Me3+ + PO43‐ → MePO4 (1.4)
The metal (Me) salts are generally added in excess to compete with natural alkalinity, according to the following reaction [
5]:
Me3+ + 3 HCO3- → Me(OH)3 + 3 CO2 (1.5)
In current decades, microalgae appear to be an ideal key as they can uptake pollutants especially nutrients in wastewaters and thereafter reproduce as a feedstock of biofuel, food and chemicals. Meanwhile, autotrophic cultivation, so far the most common way for algae, is only appropriate when CO
2 is available. And the light shading effect would restrict the high-density growth of phototrophic algae as well. By such, the cost in the heterotrophic cultivation of algae with wastewater can be greatly lessened due to the elimination of aeration, extra substrate material and sterilization. As the quantity of wastewater generated every day is enormous, it is highly possible to utilize the wastewater for microalgae cultivation [
6]. Previous studies demonstrated the success of using microalgae to treat wastewaters rich in nitrogenous and phosphorus compounds [
7-9]. In the earlier reports, isolation and characterization of tolerant microalgal strains from wastewater treatment plant influents were carried out [
7,
8,
10,
11]. Alternatively, study on screening of microalgae capable of removing nutrients and producing high quality biomass while accumulating lipids in effluents from wastewater treatment plants have been currently conducted and produced the best results [
12-17]. Currently, the research on this area is insufficient and the commercial application of biofuel based on heterotrophic microalgae cultivation is limited due to the high cost of the artificial mediums. The industrial cultivation of microalgae faces an economic feasibility issue because of the high price of artificial medium and the low levels of biomass production. However, the use of wastewaters as media can meaningfully decrease the economic cost related to the biomass for biofuel manufacture from microalgae as well as an interesting tool for wastewater treatment. This could reduce the cost of heterotrophic cultivation significantly [
18].
However, the organic substrates that have been reported for heterotrophic cultivation are restricted in a couple of categories, such as glucose, glycerol and acetate [
19]. Actually, it was economically unfeasible to culture microalgae with glucose as that cost can reach up to 80% of the total material [
20]. Thus, it is necessary to acquire more information about the adaptation of the algae in case of unusual organics rather than the preliminary differentiation as heterotrophic or autotrophic.
1.2 Overview of microalgae
1.2.1 Cultivation of microalgae
To realize the commercialization of microalgae-based bioenergy and byproducts, a number of needs must be met: (1) inexpensive culture media, (2) low cost algae production systems that can be easily installed and maintained, (3) sufficient carbon dioxide sources for optimal algae growth, (4) efficient algae harvesting methods, and (5) cost-effective and low-energy input algal lipid extraction methods. Algal growth in nutrient-rich wastewaters could help address the first requirement and, when combined with an economical algae cultivation system, could provide a cost effective supply of algal biomass to downstream processing. Most of the current algae cultivation systems can be categorized into three groups according to their reactor design: open systems, closed systems, and hybrid systems, all of which are used on land. The major difference among these systems is whether the algae are exposed to the surrounding environment. However, they also share one common feature, that is, they all utilize suspended cultures in an aquatic environment.
So far, reactors used for mass cultivation of phototrophic microorganisms (comprising microbial or algal cells) in a photobiological reaction are named Photobioreactors (PBR) [
21]. Depending on whether or not exposure to the air, PBR for microalgae cultivation can be simply classified as open systems or closed systems. The commonly used open systems in wastewater treatment plants include lagoon, high stabilization pond, aerated ponds, raceway pond, multi-layer pond-like bioreactor, etc. The most prominent features of open pond system include simple construction, low cost and simple operation. However, the disadvantages of such system are also obvious, such as large occupation area, not stable culture system, difficult to control, easy to get polluted, water evaporation loss issue and the fact that light intensity decays rapidly with medium depth. Nonetheless, from the perspective of economic viability, advanced raceway pond with paddle-wheel is considered as the most promising culture system for low cost treatment of different types of wastewaters generated from sewage, industry and agriculture as well as biofuels feedstock accumulation [
22]. It is worth noting that although most microalgae in nature are obligate photoautotrophs and their growth strictly depends on photosynthetically derived energy, there are some species that can grow in a heterotrophic mode in the presence of organic carbon under complete darkness or in a mixotrophic culture mode when supplied with both organic and inorganic carbon under light and dark cycle conditions [
23].
1.2.2 Heterotrophic cultivation of microalgae
Heterotrophy is defined as the utilization of organic compounds for growth. In a heterotrophic environment, microalgae are in competition with bacteria and can be disadvantaged by size, metabolic speed and versatility in changing environments. However, the ability to grow in the absence of light or a CO
2 supply puts these algae at an advantage over other phytoplankton species when these resources may be in limited supply. Benefits of heterotrophic growth over autotrophic growth in terms of commercial application for biofuel production include higher growth rates leading to high cell mass, protein and lipid accumulation [
24], heterotrophic microalgae as a feedstock may still provide environmental benefits that other biofuels do not, such as not being in competition for land, ability to recycle waste for nutrients and potentially wastewater treatment, and low energy inputs.
Most domestic wastewater contains organic carbon, nitrogen, phosphorous, and other minor compounds. However, both biomass production and lipid accumulation are limited by several factors of which, nutrients play a key role. Microalgae adopt various routes of metabolism for their growth and survival with autotrophic, heterotrophic and mixotrophic modes. Some microalgae can grow photoautotrophically by using light as a sole energy source that can be converted to chemical energy through photosynthetic reactions and heterotrophically by utilizing only organic compounds as both carbon and energy source and mixotrophically using both organic compounds and CO
2 as energy source [
25]. Among photoautotrophic and photoheterotrophic modes, heterotrophic cultivation of algae offers several advantages including higher growth, good control over cultivation process, minimization of light requirement and easy biomass harvesting [
26]. The heterotrophic cultivation of microalgae can bring new insight to the production of biodiesel through which algae in this mode can be cultivated in a closed system, by significantly reducing the high costs and contamination that usually occur in traditional open systems [
27].
Additionally, heterotrophic cultivation of microalgae in combination with wastewater utilization offers multiple benefits to both the energy sector and to the environment. The nutrients and the other organic compounds existing in the wastewater can serve as a source of nutrient medium for the growth of algae. The process also provides economic mode of lipid production from the treatment of wastewater. Besides growing algal biomass for biofuel use, the wastewater can be treated simultaneously. Thus, the double benefits have attracted extensive attention over the years. But this approach has its shortcomings: (1) some wastewater may be too toxic to support algal growth; (2) due to the outdoor nature, algal growth and wastewater treatment efficiency can be significantly affected by seasonal alterations; and (3) competition among the microbial community in the wastewater may make algal growth very slow. Hence, using microalgae to treat wastewater while expecting high biomass productivity, it can be problematic. Furthermore, to overcome these problems, several research groups have attempted to screen microalgal species that can be dedicated for this dual-process. One example is to select algal strains that are facultative heterotrophic, adaptable to northern climate, able to consume organic carbon, nitrogen, and phosphorous in wastewater, and capable of high yield of biomass and lipid [
28].
1.2.3 Microalgal harvesting
After microalgal growth, the important step is to harvest the biomass. Harvesting microalgal cells from growth culture is a significant challenge in many industries for microalgal biomass production. Currently, the biomass separation and harvesting has posed another tough problem in the field of the algal cultivation, no matter for microalgae production or wastewater treatment. During microalgal cultivation in wastewater treatment systems that employ gravity thickening, filtration, secondary settling tank, and secondary clarifier for separating water and sludge (or biomass), the suitable cost-effective procedure has to be implemented in order to harvest the microalgal biomass prior to biofuel conversion [
29].
Also, the choice of harvesting method is significantly dependent on the economic factors, harvesting efficiency, microalgae species and desired quality of the products [
29]. For chemical harvesting technique, usually chemical flocculation is induced by specific electrolytes, synthetic polymers, organic flocculants and inorganic are normally employed [
30]. For mechanical methods, sedimentation method is used to harvest microalgal cells [
31], filtration, dissolved air flotation [
32,
33], using ultrafiltration membranes and attached algal biofilms [
32], and then centrifugation [
34]. Agreeing to Greenwell et al., [
33], the key factor that limit the commercial use of microalgae as one of the most challenging areas in the algal biofuel research, is the development of a cost effective harvesting method which can reach up to 20–30% of total production cost [
35].
Besides, filtration and centrifugation requires high energy input for operation which would be expensive. Conversely, studies showed that the application of commercial polymers at different concentrations for harvesting algae-bacterial flocs and reported that the flocculation efficiency decreases within increase in concentration of polymers [
36]. Recently, microalgae harvest educing nanoparticles (ex: Fe
3O
4) under in situ magnetic separation yielded maximal recovery efficiency more than 98% at a stirring speed of 120 rpm, with nanoparticles resulted in 55.9 mg-dry biomass mg-particles
-1 for
Botryococcus braunii and 5.83 mg-dry biomass mg-particles
-1 for
Chlorella ellipsoidea [
37]. Organoclays (aluminium and magnesium based amino salines clay) have been effectively used for microalgae harvesting with 100% efficiency in 30 min under neutral pH [
36]. In addition, filtration is a process that separated solid and liquid phases with the help of membrane. This process does not require any chemical agents for harvesting. The major limitation is biofouling of microbes in to the membrane of the filter; in order to overcome anti-fouling, strategies are implemented for fast processing. Dynamic filtration with pH induced flocculation is considered to be effective for more biomass production in open ponds [
38].
Gravity sedimentation is a simple method commonly applied in water and wastewater treatment to separate solids. The rationale of the process is to use gravity to separate liquids and/or solids from another liquid with different densities. Nevertheless it is an easy and simple method, it has several drawbacks since it is time and space consuming and the separation of low density microalgal particles is often unsuccessful [
39]. Centrifugation is a highly efficient and reliable method, where most microalgae particles can be recovered from the liquid cultures, with about 95 - 100% and 80 - 90% efficiency using centrifugation at 13,000 g and at 500 - 1,000 g, respectively [
40]. This is an effective method when dealing with the relatively low biomass concentrations obtained from microalgal cultivation systems with just a slight difference in density with respect to the liquid phase and the small size of microalgal cells. An oil extraction/trans-esterification with 90% efficiency would give 1.89 kg of fatty acid methyl esters (FAMES) containing 19.8kWh. Thus, the centrifugation operation alone would consume 49 kWh to produce only 19.849 kWh which is far from being sustainable [
41]. Bio-flocculants have emerged as an innovative technological approach in flocculation technology in order to minimize the energy consumption of harvesting microalgae. Bio-flocculation methods are flocculation induced by extracellular polymer compounds such as polysaccharides and proteins, derived from microalgae and other microorganisms [
42]. Various latest technologies for harvesting of algal biomass are recently reviewed [
42-44]. Flocculation is an enhanced process of sedimentation, with the addition of flocculants to improve the biomass settling rate.
Flocculation is similarly known as the process where the dispersed particles in suspension are aggregated together by the addition of chemicals (flocculants) to form larger particles that can easily settle. On behalf of harvesting purposes the dispersion induced by the electric charge increases the difficulty of separating the biomass from the water. The use of flocculants to surpass this characteristic is an efficient and common solution widely used in similar applications (e.g. wastewater treatment systems) and has been shown to be efficient with microalgae as well. The rational is to use positively charged ions or polymers (e.g. FeCl
3, Al
2(SO
4)
3, Fe
2(SO
4)
3 or natural and starch derivatives), which aid coagulation and improve algal biomass settling.
In
Mara [45], it is shown that the chemical flocculants used either inorganic or organic vary in effectiveness depending on their ionic charge and algal strain, but a report claimed to achieve up to 80% harvesting efficiency using pH induced Fe
3+ flocculation for various algal species. Chitosan as an important flocculants, by-product of shrimp and crab industries produced from the chitin of these animals is currently being tested in different industries such as chemical, food, pharmaceutical and in agriculture. In Riano et al., a non-toxic and biodegradable polycationic polymer has shown promising results as microalgae flocculant [
46]. The typical flocculants should be effective in low concentrations, low cost, non-toxic and have no effect on medium recycling (e.g., polyelectrolytes, aluminium sulphate and ferric chloride [
35]. To this, during chemical flocculation, microalgal harvesting involves bioflocculation, whereby microalgae spontaneously flocculate with bacteria and settle in the ponds. Microalgae-bacterial flocs from secondary treatment sewage can remove 97.5% of biomass from the culture medium in 30 min [
41]. In contrast, flocculation in large scale pond systems, was reported as ineffective harvesting method [
47].
Moreover, the choice of biomass harvesting method is mainly driven by economics and strictly depends on the value of the product. For example, gravity sedimentation possibly enhanced by flocculation, may be used for low value products and sewage based processes [
48], while high value products, such as those for food, feed and nutraceuticals may permit the use of cost intensive continuous centrifuges. Indeed, there is no universal best method for microalgal harvesting and mainly depends on algae species, size, density, production costs, growth medium and the end product. It was demonstrated that a universal harvesting method does not exist but in order to concentrate large microalgae culture volumes, a suitable harvesting method may involve one or more steps and be achieved in several physical, chemical, or biological ways [
49].
Therefore, according to Gutiérrez et al. [
50], the commonly employed biomass harvesting techniques (e.g. flocculation, filtration, centrifugation etc.), may account for up to 20-30% of the total cost in microalgae production. Thus, promoting the spontaneous flocculation and gravity sedimentation by increasing the dominance of the self-settleable microalgae rose as an appealing strategy for algae harvest in recent years [
51]. In this sense, microalgal strains with high self-settleability are of great interest in any cultivation system.
1.3 Cultivation of microalgae in wastewater
1.3.1 Sampling and isolation of microalgae from natural environment
Microalgae are normally found in aquatic bodies including freshwater, marine ecosystems and brackish water systems. Microalgae grow in most of the natural environments including water, rocks and soil, but interestingly also grow on and in other organisms. Local microalgae species should be collected because it can be expected to have a competitive advantage under the local geographical, climatic and ecological conditions. Microalgae may also be found in environmental conditions such as hot spring lakes, acid mine drainage and ice-lakes [
52,
53]. Some microalgal strains can grow even on land such as rocks and ionizing radiation fields such as nuclear reactors [
54], whereas some microalgae are capable to display only in brine and require saturated salts for their growth [
54]. Cyanobacteria, considered as microalgal, are photosynthetic prokaryotes often found in normal as well as in extreme environments, forming thick microbial mat during their growth and are found in extreme environments, such as hypersaline aquatic environments, dry and hot deserts, and sub-zero temperatures in the polar regions [
55]. Nubel et al., have isolated six different strains of cyanobacteria belonging to the genera of spirulina, which are tolerant and able to survive at a high temperatures [
56].
Some other microalgal species (e.g.,
Chlorella sp.,
Euglena mutabilis and
Pinnularia sp.) exhibit photo-inhibition and typically located at the bottom of the river bed [
54],
Massimi and Kirkwood [57] isolated forty-three algal strains including several strains from natural habitats with the exception of one cyanobacterial strain and Chlorophyta [41]. Nevertheless most microalgae are photoautotrophic with a great diversity in the natural habitat and can be found and collected from lakes, ocean, river, pond, and other water bodies [
58]. Isolation is a necessary process to obtain pure cultures and presents the first step towards the selection of microalgae strains with potential for biodiesel production. After collecting samples, methods to isolate axenic cultures are essential to get rid of other unwanted microorganisms (e.g. bacteria, fungus) through series of dilutions, sub-culturing, plating and anti-bacterial procedure.
Isolation of microalgae is normally practiced by three different plating methods, which are popularly known as streak plate, spread plate, and pour plate methods [
54,
59]. Streaking method is accomplished by streaking the loop containing algal sample over the agar surface and the numbers of algal cells are reduced at the end of the streaking helping to form a single colony. Pour plate method is done in case of those microalgae which don’t grow on the surface of the agar. In pour plate, direct dilution of algae is achieved while being suspended in the poured agar, ensuring separation at the time of plating. In addition, solidifying substance of the liquid medium containing microalgal cells traps the individual cells in place. In agar medium, they produce a fixed colony of cells or filaments and grow as separate colonies. In spread plate method, the microalgal culture is dispersed all over the surface of solid media, which leads to the isolation of the independent colonies [
55].
There are several other techniques available. One simple but effective method for isolation is the serial dilution method. It is a common technique used to bring down the concentration of microscopic organisms or cells in a liquid sample. By using this technique, an axenic culture may grow in one of the higher dilution tubes [
55]. Pure culture may also be obtained using picking up method (capillary method), which follows the dilution method, but inoculum is prepared by selecting single cells of the desired species by operating a capillary pipette handled under a microscope [
60]. Another effective method for isolation is Fluorescence Activated Cell Sorting (FACS) using Flow Cytometer enabling the selection of specific strains of microalgae and subsequent isolation [
61].
Another cost effective and high-throughput isolation and identification method for marine microalgae has been reported. This system consolidated high throughput isolation by streaking cells from enriched cultures on agar plates with subsequent development in multi-well plates [
62]. Satpati et al., reported that approximately 150,000 different microalgal species have already been identified from different habitats and many more are yet to be identified and to be included in the database [
63]. To this, more than 50% of identified algal species are from the marine and approximately 40% are from the freshwaters while 99% screened from aquatic bodies mainly belongs to
Chlorophyceae,
Bacillariophyceae,
Chrysophyceae,
Euglenophyceae, etc. [
63]. Also, each microalgae strain requires careful selection and optimization in order to increase biomass and lipid productivity with the aim to provide a cropping system with improved biofuel production and performance properties [
64].
1.3.2 Isolation of microalgae from natural environment
Previous studies are mainly focused on several locally isolated microalgal strains such as
Chlorella [
65]. Heterotrophic cultivation of algae with wastewater still faces many obstacles in practice. The primary problem is that there is a limited number of microalgal species that can grow heterotrophically. Indeed, most known microalgae species are obligate autotrophs instead of heterotrophs or mixotrophs [
66]. However, researchers tried to isolate and cultivate the heterotrophic microalgae in various categories of wastewaters. Tian-Yuan et al. [
67] isolated 5 heterotrophic microalgae from a domestic wastewater treatment plant and heterotrophic metabolism of isolates were evaluated for biofuel production using the Biolog method. Wang et al. [
68] isolated
Chlorella sp. from local wastewater, cultured it in different MWWs and observed significant heterotrophic growth rates and nutrient removal. Zhou et al. [
9] realized the heterotrophic cultivation of microalgae in concentrated municipal wastewater (CMWW), Ummalyma and Sukumaran [
69] used heterotrophic microalgae to treat dairy effluent for oil production and organic removal. Also Zhang et al. [
7] treated real wastewater using the newly isolated heterotrophic microalgae where the algal densities of
Scenedesmus and
Chlorella species were increased by 203.0% and 60.5%, respectively while Ramsundar et al. [
70] assessed the potential of
Chlorella sorokiniana in MWWs at various stages of treatment.
Currently, only few heterotrophic microalgae species have been isolated and a little work has been carried out in real wastewater treatment by heterotrophic microalgae without additional nutrients supplementation. In order to move forward the prospects of large-scale cultivation of microalgae in wastewater, potential microalgae isolated from local wastewater treatment plants to serve as candidates for pollutants removal and biofuel production are needed. The rationale for this is that microalgal isolates would have inherently higher tolerance to wastewater compared to isolates from natural systems, as well as an increased capacity to grow heterotrophically to exploit wide range of organic substrates in wastewaters.
Another challenging task to the researchers worldwide is the morphological identification of interesting algae as it lacks precise morphological markers for identification. Microalgae can change the cell size and shape during different stages of their life cycle; hence, it requires great effort to analyze the microalgae by conventional microscopic techniques [
71]. Morphological identification of microalgae requires experienced, taxonomical and technical expertise. Molecular identification tools can complement microscopic identification, and vastly improve the reliability of taxonomic classification of the identified algae. Molecular identification by ribosomal DNA sequencing is rapid, accurate and has proved to be very useful in understanding the evolutionary relationship among different species of algae [
72].
1.3.3 Nutrients requirements
Carbon, Nitrogen and Phosphorous are key components for the algae for nutrient assimilation. The nitrogen mainly comes from the sewage by the metabolic conversion and more than 50% phosphorous comes from the detergents. The principal forms in which they occur in wastewater are NH
3 (ammonia), NO
2 (nitrite), NO
3 (nitrate) and PO
4 (orthophosphate). From Perez-Garcia et al. [
73], these two elements are known as nutrients and their removal is known as nutrient stripping.
The rapid development of human activities has greatly increased the input of nitrogen and phosphorus into bodies of water. This input induces eutrophication and causes deterioration in natural water quality. As such, the removal of nitrogen and phosphorus from wastewater is a fundamental way to prevent eutrophication and water bloom. The main pollutants in a variety of wastewater sources are nitrogen and phosphorus in different forms, which contribute to eutrophication of water-bodies (e.g. lakes, rivers or oceans worldwide) if discharged improperly. On the other hand, these pollutants are ideal nutrients to stimulate fast algae growth. All organisms require basic nutrients for growth and multiplication, and most microalgae can meet all their cellular needs for their growth with a few key compounds; macronutrients, micronutrients (trace elements) and vitamins [
74]. Jimenez-Perez et al. [
75] reported that microalgae isolated from wastewater treatment plant sites or real bodies of water can adapt to practical conditions better and grow well. Cultivation of microalgae system in wastewater helps to reduce the organics by synthesizing biomass production and there by preserving fresh water resources [
76]. Thus the main mechanisms for nutrients removal in wastewater by microalgae include typical photosynthetic assimilation and chemosynthetic assimilation by heterotrophic or mixotrophic metabolic pathway.
1.3.4 Microalgae-based wastewater treatment
1.3.4.1 Nitrogen removal in algal cultures
Nitrogen is the most important nutrient contributing to the algal biomass production and is a key constituent of many algal cellular components such as amino acids, DNA, RNA proteins, alkaloids, vitamins, amides, enzymes and hormone accounting for 1-10% of dry cell weights, depending on the supply and availability [
77,
78]. Furthermost microalgae species are able of employing a variability of nitrogen sources comprising organic nitrogen (e.g., peptone, yeast extract, urea, glycine, glutamine, etc.) and inorganic nitrogen (e.g., nitrate, nitrite and ammonia, etc.) [
77]. It has been reported that nitrogen metabolism is related to carbon metabolism in microalgae because they share organic carbon and energy supplied directly from photosynthetic electron transport and CO
2 fixation as well as from the metabolic pathway of organic carbon through heterotrophic cultivation [
77]. For instance, assimilation and uptake of inorganic nitrogen (e.g., nitrate) by algae to form amino acids requires carbon skeletons in the form of keto-acids (2-oxalogutarate and oxaloacetate) and energy in the form of ATP and NADPH to synthesize the glutamine and amino acids glutamate [
79]. Among the above nitrogen forms, ammonium is the most preferred form of nitrogen source for microalgae in part because its uptake and utilization by microalgae is most energy-efficient [
77]. From above discussion, it is obvious that the preference for microalgae nitrogen uptake and assimilation by most microalgae species is: ammonium > nitrate > nitrite > urea [
77]. Nitrogen removal from wastewater treatment processes is mainly achieved by algal cells assimilation.
1.3.4.2 Phosphorous removal in algal cultures
Microalgae, like all organisms, need phosphorus to grow. The phosphorus is used in the algal cells mostly for phospholipids production, nucleic acids and ATP. Likewise, microlgae assimilate phosphorus as inorganic orthophosphate, possibly as H2PO4‐ or HPO42-. Phosphorous (demonstrating about 1-3% of microalgae in dry cell weights), as a major macronutrient, plays a significant role in cellular metabolic routes including production of nucleic acids, DNA, energy transfer and probably forms various functional constituents and structures essential for development and growth [
21]. Then, orthophosphate is merged into organic compounds through several kinds of phosphorylation procedures. Growing algae in phosphorus rich wastewaters could display the competent uptake of phosphorus stored in polyphosphate bodies influenced with a multiplicity of factors such as temperature, phosphate, light intensity and concentration in wastewater.
Nevertheless, the mechanisms of how these factors affect luxury uptake are not fully agreed [
21]. The ratio of N:P also plays an important part in N and P removal in algal based wastewater treatment system as it not only determines the potential productivity but also is important in maintaining the dominance of candidate species in culture [
21]. The inorganic N:P ratio varies with different types of wastewaters. A range of approximately 6.8-10 is considered as the optimal N:P ratio for algae growth. A ratio of N:P much higher than the optimal ratio indicates a high probability of phosphorus limitation while N:P ratio much lower than the optimal ratio indicates a high probability of nitrogen limitation [
80]. Apart from photosynthetic and chemosynthetic assimilation by microalgae, N and P removal from wastewaters are also affected by abiotic factors significantly. For example, at high pH, ammonia could be easily removed through stripping and phosphorous could be coagulated with metal ions that naturally existed in wastewaters and removed through precipitation [
81]. Therefore, the N and P removal in wastewater based algal cultivation system were attributed to assimilation by microalgae as well as volatilization and precipitation caused by abiotic factors.
1.3.4.3 Organic pollutant removal in algal cultures
Numerous microalgae are capable of growing photoautotrophically using inorganic carbon and sunlight as carbon and energy sources, respectively, heterotrophically using organic compounds as carbon and energy source, and mixotrophically by combining phototrophic and heterotrophic metabolisms [
19]. To this, microalgae synergistically interact with aerobic heterotrophs and autotrophs via exchange of substrates. These metabolic abilities and interactions support the biodegradation of biodegradable organic pollutants, energy use and its associated impacts, and reduce the risks of spreading of hazardous pollutants and pathogens [
82].
Carbon is one of the important sources for microalgae for cell growth as inorganic carbon dioxide, in autotrophic mode of cultivation. It would be organic carbon source for heterotrophic and mixotrophic mode of cultivation. Heterotrophic cultivation of microalgae has been reported to provide not only a high cellular oil content, but also a high microalgal biomass productivity [
83]. So, heterotrophic cultivation of algae suggests a promising way in the circumstance of wastewaters, in which organic carbon can be simultaneously used as energy and carbon source. By such, the cost in the heterotrophic cultivation of algae with wastewater can be greatly lessened due to the elimination of aeration, extra substrate material and sterilization. Additionally, the operation and daily maintenance turn to be much simpler than the autotrophic method. With all these merits, heterotrophic cultivation has been reported to enhance the algal biomass yield up to 25-fold of the autotrophic mode [
84]. Although, in the process of fixation, microalgae use CO
2 as an inorganic carbon source, while water acts as an electron donor for production of glucose, which is further transformed to various complex sugar forms such as carbohydrate, starch etc. Many microalgae species are able to utilize carbonates such as sodium carbonate (Na
2CO
3) and sodium hydrogen carbonate (NaHCO
3) for cell growth [
85]. Some studies have indicated that about 25–50 % of the algal carbon in high rate algal ponds is derived from heterotrophic utilization of organic carbon. The organic carbon sources can be assimilated either chemo- or photoheterotrophically. In the first case, the organic substrate is mused both as the source of energy (through respiration) and as carbon source, while in the second case, light is the energy source. In several algal species, the mode of carbon nutrition can be shifted from autotrophy to heterotrophy when the carbon source is changed; this is the case with e.g. of the green algae
Chlorella and
Scenedesmus [
74].
1.3.5 Microalgae biomass production in wastewater
Fast urbanization worldwide impacts the intensification in usage of water means in daily accomplishments which lead to depletion of freshwater resources. According to this statement, wastewater treatment has been mainly implemented widely to reuse the wastewater in order to conserve the available freshwater. Wastewater is a potential support for the life of various organisms as it contains more organic and inorganic compounds. Among the wastewater sources (i.e., industries, agricultural and domestic etc.), municipal wastewater has been showed a potential microalgal growth [
36]. Preceding investigators have considered the cultivation of microalgae in domestic wastewater collected from several wastewater treatment plants [
86,
87]. In those reports, the microalgae strains were suitable to produce high density biomass for multiple applications. From Li et al., it is highly possible to utilize the wastewater for microalgae cultivation, as the quantity of wastewater generated every day is huge [
6]. The microalgal cultivation conditions as reported by Kovar et al., may be photoautotrophic (where sunlight produces energy and transforms inorganic ingredients into organic ones), mixotrophic (assimilation of organic carbon substrates accompanied by inorganic compounds in light existence) and heterotrophic (unable to synthesize its own organic carbon compounds from inorganic sources and use organic matter as carbon source and energy simultaneously from other organisms) [
88].
However, the isolation of microalgae capable of high biomass and lipid concentration production is the foundation for biofuel development. Therefore, effective mutual advanced wastewater treatment and biomass production, selecting the proper microalgae species is the key point of research in this field. It has been reported that heterotrophic cultivation of microalgae is an effective way to support a much higher density of microalgae biomass than autotrophic cultivation [
89], it has been realistic in the large-scale production for several years ago [
90], it could also decline the land area needed and water evaporation significantly [
91]. In addition, heterotrophic cultivation of microalgae, glucose [
92] and acetate [
93] have been successfully used as carbon source whereas the type and concentration of nutrient sources were also supplemented to the medium [
92]. Thus, the heterotrophic cultivation of microalgae using wastewaters is a promising way to improve the feasibility of large-scale application of microalgae in biomass production for biofuels.
1.3.6 Biofuel production in wastewater
Biodiesel production from microalgae has received special attention as it contains hydrocarbon in the form of lipids, which is the most important criteria for biodiesel production. Nitrogen shortage in growth culture supports microalgae to accumulate more lipids in cells but in contrast, growth of cells was limited due to fewer nutrients in the medium [
94]. Previously, several studies attempted to use green microalgae in numerous wastewaters to generate biodiesel forms such as fatty acids and lipid. For example, in sewage,
Chlorella species produces 0.12 g biodiesel L
-1 [
81],
Chlorella ellipsoidea YJ-I accumulates 11.4 mg L
-1 of lipid content [
86],
Scenedesmus AMDD yields approximately 10 – 18 mg FAMES L
-1 day
-1;
Chlorella sp
. produces 6.9 mg lipid L
-1 d
-1 [
95]. When using industrial wastewater like citric acid effluents,
Chlorella vulgaris C9-JN 2010 reached 342 mg g
-1 lipid. In piggery wastewater,
Chlamydomonas mexicana produces 0.03 g lipid L
-1,
Chlorella pyrenoidosa harvests 6.3 mg lipid L
-1 d
-1,
Chlorella vulgaris produces 0.07 g lipid L
-1,
Botryococcus braunii produces 0.95 g hydrocarbon L
-1, whereas, in dairy wastewater,
Chlorella pyrenoidosa earnings 6.5 mL biodiesel while in soya bean wastewater,
Chlorella pyrenoidosa revenues 0.35 – 0.54 g lipid L
-1 d
-1.
Moreover, microalgal cultivation in collective wastewater from carpet mill industry and sewage produces 63.9% of oil from microalgae which can be converted in biodiesel yield. The involvement of diverse wastewater may prevent overwhelm of the growth of microbe due to certain constituents present in wastewater. Apart from the lipid content, microalgae also possess carbohydrates content which can be used for synthesis of bioethanol production. Also,
Scenedesmus species cultured in a raceway pond reactor produces 29% of sugar with dark/light cycle. Though, utilization of enzymes for cultivation of
Chlamydomonas reinhardtii for manufacturing ethanol using yeast produces 235 mg of ethanol from 1g of microalgal biomass. Under discrete hydrolysis fermentation,
Chlorella vulgaris FSPE produced 11.7 g ethanol L
-1 [
94].
1.4 Objectives of the study
1.4.1 Main objective
The purpose of this study is to screen the isolated microalgal strains from domestic wastewater treatment plant aimed at algal biomass production under heterotrophic cultivation. It aims in reviewing the potential of newly isolated strains with the high heterotrophic growth capacity to assimilate nutrients and biomass production using domestic wastewater as a resource.
1.4.2 Specific objectives
The specific objectives of this study were:
(1) To screen algae from domestic wastewater.
(2) To characterize isolated microalgal strains.
(3) To evaluate their performance in terms of high-dense growth, organics tolerance, nutrient removal, lipid recovery and self-settleability.
(4) To select suitable microalgae strains to realize the heterotrophic cultivation with real domestic wastewater.
(5) To evaluate the effects of selected microalgal strains on the environmental factors (Temperature and pH).
(6) To evaluate the effects of carbon sources on selected microalgal isolates.
Chapter 2 Materials and Methods
2.1 Equipment and Reagents
2.1.1 Equipment
The following apparatuses were used throughout this study:
Table 2.1 Equipment
Serial No. |
Name |
Model |
Manufacturer |
1 |
Analytical balance |
Practum 224-1CN |
Sartorius (Germany) |
2 |
Autoclave |
HIclave TM HV-119 |
HIMALAYA (Japan) |
3
4 |
Centrifuge
Centrifuge |
Ankle TGL-16B |
Hunan XINKE Scientific Instruments Co., Ltd, (China) |
5804 R |
Eppendorf (USA) |
5 |
Shaker incubator |
SKY-2102C |
SUKUN (China) |
6 |
Microscope |
DM500 |
Leica (Switzerland) |
7 |
Freeze dryer |
FDU-1200 |
EYELA (Germany) |
8 |
Vortex shaker |
WH-861 |
SMT Max Corporation (USA) |
9 |
pH meter |
Delta 320 |
Mettler Teledo (China) |
10 |
Spectrophotometer |
UV-1780 |
Shimadzu, (Japan) |
11 |
TOC Analyzer |
TOC-L CPH |
Shimadzu (Japan) |
12 |
Microplate Reader |
SpectraMax 190 |
Molecular devices (USA) |
13 |
Oven |
DHG-9070A |
SHINOVA (China) |
14 |
Centrifuge tubes |
NEST |
NEST Biotechnology Co., Ltd. (China) |
15 |
Petri dishes |
MCD-000-090 |
JET BIOFIL (China) |
16 |
Microplates |
NunclonTM |
Thermo Fisher Scientific (China) |
2.1.2 Reagents
The following reagents were used throughout this study:
Table 2.2 Reagents
Serial No. |
Reagent name |
Design |
Manufacturer |
1 |
Glucose |
AR |
Sinopharm Chemical reagent Co., Ltd. |
2 |
NaNO3 |
AR |
Sinopharm Chemical reagent Co., Ltd. |
3 |
K2HPO4.3H2O |
AR |
Sinopharm Chemical reagent Co., Ltd. |
4 |
KH2PO4.3H2O |
AR |
Sinopharm Chemical reagent Co., Ltd. |
5 |
MgSO4.7H2O |
AR |
Sinopharm Chemical reagent Co., Ltd. |
6 |
Ferric ammonium citrate |
AR |
Sinopharm Chemical reagent Co., Ltd. |
7 |
H3PO4 |
AR |
Sinopharm Chemical reagent Co., Ltd. |
8 |
HCL |
AR |
Sinopharm Chemical reagent Co., Ltd. |
9 |
EDTANa2 |
AR |
Sinopharm Chemical reagent Co., Ltd. |
10 |
Glycerol |
AR |
Sinopharm Chemical reagent Co., Ltd. |
11 |
Citric acid |
AR |
Sinopharm Chemical reagent Co., Ltd. |
12 |
Agar |
BR |
Solarbio Science & Technology Co., Ltd. |
13 |
Na2CO3 |
AR |
Sinopharm Chemical reagent Co., Ltd. |
14 |
CaCl2.2H2O |
AR |
Sinopharm Chemical reagent Co., Ltd. |
15 |
NaCl |
AR |
Guandong Guanhua Sci-Tech Co., Ltd. |
16 |
NaOH |
AR |
Guandong Guanhua Sci-Tech Co., Ltd. |
17 |
Methanol |
HPLC |
Sinopharm Chemical reagent Co., Ltd. |
18 |
Soluble starch |
AR |
Sinopharm Chemical reagent Co., Ltd. |
19 |
MgSO4.4H2O |
AR |
Sinopharm Chemical reagent Co., Ltd. |
20 |
ZnSO4.2H2O |
AR |
Sinopharm Chemical reagent Co., Ltd. |
21 |
Na2MoO4.2H2O |
AR |
Sinopharm Chemical reagent Co., Ltd. |
22 |
CaSO4.5H20 |
AR |
Sinopharm Chemical reagent Co., Ltd. |
23 |
Co(NO3)2.6H2O |
AR |
Sinopharm Chemical reagent Co., Ltd. |
24 |
Xylose |
AR |
Sinopharm Chemical reagent Co., Ltd. |
25 |
Na-acetate |
AR |
Sinopharm Chemical reagent Co., Ltd. |
26 |
α-Lactose |
AR |
Sinopharm Chemical reagent Co., Ltd. |
27 |
Glycerol |
AR |
Sinopharm Chemical reagent Co., Ltd. |
28 |
D-Galactose |
AR |
Sinopharm Chemical reagent Co., Ltd. |
29 |
Puruvuc acid |
AR |
Sinopharm Chemical reagent Co., Ltd. |
30 |
DL-Malic acid |
AR |
Sinopharm Chemical reagent Co., Ltd. |
31 |
Maleic acid |
AR |
Sinopharm Chemical reagent Co., Ltd. |
32 |
L-Glutamic acid |
AR |
Sinopharm Chemical reagent Co., Ltd. |
2.2 Sampling site and wastewaters
In this study, the mixed liquor in the aeration tank was sampled in Xiapu Wastewater Treatment Plant in Xiamen, China. After settling, the pellet was used as the original source for algae screening, and the supernatant was used as the real (without any other sources of substrates) domestic wastewater (RDWW) in the treatment experiment. All the samples were placed into a cooler and stored at 4
˚C or -20
˚C to avoid variation of wastewater composition.
Table 2.3 Media used in isolating microalgal strains and concentration of chemical components
Component |
Concentration in media |
MBG11 (mg L-1) |
MBold-3N (mg L-1) |
NaNO3 |
82.57 |
82.57 |
K2HPO4 |
12.46 |
12.46 |
KH2PO4 |
- |
88 |
MgSO4 .7 H2O |
75 |
75 |
CaCl2·2H2O |
36 |
25 |
Citric acid |
6 |
- |
Ferric ammonium citrate |
06 |
- |
FeCl2.6H2O |
- |
1770 |
EDTA (disodium salt) |
1 |
2440 |
Na2CO3 |
20 |
- |
H3BO3 |
2860 |
- |
MnCl2·4H2O |
1810 |
- |
MnSO4.5H2O |
- |
0.589 |
ZnSO4·7H2O |
222 |
0.073 |
Na2MoO4·2H2O |
390 |
0.00148 |
CuSO2·5H2O |
79 |
- |
Co(SO4)2·7H2O |
- |
0.016 |
Co(NO3)2·6H2O |
494 |
- |
NaCl |
- |
25 |
NiCl2.6H2O |
- |
0.00149 |
2.3 Isolation of microalgal strains
The isolation method was modified from Wang et al. [
7]. Briefly, 100 mL of wastewater samples were placed into sterile 250 Erlenmeyer flasks (triplicate) and placed on continuous light bank to encourage algal growth in whole-effluent for 30 hours.
Fig. 2.1 Process diagram for isolation strategy of heterotrophic microalgal
When growth was confirmed either by naked eye or microscopic examination, 4 mL of wastewater sample was transferred to 250 mL flask containing either modified blue green (MBG11) [
96] or modified Bold-3N media (MBold-3N) [
97] in each medium. Compositions of isolation media were shown in Table 2.3.
After growth establishment in media, 2 mL of culture were added to fresh media and grown for 10 days. This process was repeated two successive times to ensure the compatibility of the algal culture under laboratory conditions. The algae were subjected to purification by serial dilutions followed by inoculation onto Agar plates containing flesh medium solidified by 12 g L
−1 of agar (triplicate). Two types of media were chosen in an attempt to isolate a larger diversity of isolates (Table 2.3).
Nitrate and phosphate were used as the nitrogen and phosphorus sources, respectively. The contents of nitrogen (NO
3-N) and phosphorus (PO
4-P) in the media were set as 13.6 mg L
-1 and 2.22 mg L
-1, respectively. Two types of media were chosen in an attempt to isolate a broader diversity of isolates. To avoid the contamination of bacteria, the citric acid was added. The purity of the cultures was ensured by repeated streaking on the nutrient agar plates and repetitive microscopic examination. Morphologically, unique strains were selected and maintained for further experimentation (Leica DM500, Switzerland).
Microscopic checking also allowed us to monitor the isolation process to ensure the presence of a single strain culture. Microscopic observation of these microalgal strains indicated their colonial existence and purity. The preservation conditions used to isolate the microalgal strains were 260 μmol photon m
−2 s
−1, light/dark ratio was 9:15 hour, initial pH 7 and the temperature range of 25 - 28
˚C for 15 days of incubation. The overall isolation route of heterotrophic microalgae was shown in Fig. 2.1 and each step was carried out in triplicate.
During the growth periods, the cultures were manually shaken 3 times per day to avoid sticking. In order to obtain the hopeful microalgal strains in biomass production for lipid production and nutrients removal from wastewaters, all isolates were grown in MBG11 medium for 10 days under dark cultivation. After this, the top growing isolates were cultured to assess their biomass productivity, nitrate concentration, phosphate concentration, residual total organic carbon and total lipid content. In addition, selection of the optimal strains was also performed (Fig. 2.2).
Fig. 2.2 Selection process of the most promising candidates among the isolates strains
2.4 Identification of microalgal isolates
2.4.1 Molecular Characterization and identification of isolated microalgae
Morphological identification of algae of interest is a challenging task to the researchers worldwide as it lacks precise morphological markers for identification. Microalgae can change the cell size and shape during different stages of their life cycle. Hence, it requires great effort to analyze the microalgae by conventional microscopic techniques and also obliges experienced, taxonomical and technical expertise. Molecular identification tools can complement microscopic identification, and vastly improve the reliability of taxonomic classification of the identified algae. Molecular identification by ribosomal DNA sequencing is rapid, accurate and has proved to be very useful in understanding the evolutionary relationship among different species of algae [
71,
98]. Identification of algal taxonomy is normally based on microscopic characteristics particularly morphology and color. There are a number of situations in which molecular techniques have taken priority, such as: No clear morphological characteristics are available (for unicellular blue-green algae), when algae are relatively inaccessible and difficult to visualize (case for biofilms or a very heterogeneous community of organisms), when diversity is being studied within species (when strains are often distinguished in biochemical and genetic terms). Finally, species proof of identity in both prokaryote and eukaryote algae may depend on molecular analysis, with determination of unique and defining DNA sequences followed by development of species-specific nucleotide probes [
99].
2.4.2 DNA extraction
Apart from the microscopic examination, molecular identification can improve the consistency of taxonomic classification of the targeted algae. In this work, the total cellular DNA was extracted from the microalgal isolates by using DNA Isolation Kit (MO BIO Laboratories, Inc., USA) based on the manufacturer's instructions. The genomic DNA was stored at -20 ˚C until PCR amplification.
2.4.3 PCR amplification and gene sequencing
The polymerase chain reaction (PCR) is a powerful and sensitive technique which amplifies specific DNA sequences [
100]. It has been reported that the 16S rRNA gene can be considered as molecular marker more effective in identifying some species of microalgae [
101]. Currently, analysis of the corresponding 23s rRNA sequences provided more information concerning phylogenetic relationships. 23s rRNA is about twice as big as 16s rRNA, so it is assumed that 23s rRNA contains more variable regions and hence is more discriminative than the smaller 16s rRNA. There has been renewed interest in the use of the 23S rRNA gene with the decrease in sequencing costs and the growing popularity of techniques such as microarrays [
102] analysis of the 16S-23S inter-genetic region, fluorescence in situ hybridization, and quantitative PCR. Compared to 16S rRNA genes, 23S rRNA genes contain more characteristic sequence stretches due to a greater length, unique insertions and/or deletions, and possibly better phylogenetic resolution because of higher sequence variation [
103].
A recent study indicated that 23S rRNA genes also contain conserved regions for designing broad-range primers with a similar degree of universality to the broad-range primers for 16S rRNA genes [
102]. There has been renewed interest in the use of the 23S rRNA gene with the decrease in sequencing costs and the growing popularity of techniques such as microarrays [
102] analysis of the 16S-23S inter-genetic region, fluorescence in situ hybridization, and quantitative PCR. Compared to 16S rRNA genes, 23S rRNA genes contain more characteristic sequence stretches due to a greater length, unique insertions and/or deletions, and possibly better phylogenetic resolution because of higher sequence variation [
103]. In the other hand, 18S rRNA gene is one of the most frequently used genes in phylogenetic studies and an important marker for random target PCR in environmental biodiversity screening [
104]. The S in 18S represents
Svedberg units. 18S rRNA is a component of the small eukaryotic ribosomal subunit, the structural RNA for the small component of eukaryotic cytoplasmic ribosomes, and thus one of the basic components of all eukaryotic cells. It is the eukaryotic nuclear homologue of 16S ribosomal RNA in Prokaryotes and mitochondria. The 18S gene is part of the ribosomal functional core and is exposed to similar selective forces in all living beings.
In this study, taxonomic determination of isolates was confirmed by sequencing using two oligonucleotide primers (Forward: 5'-GTAGTCATATGCTTGTCTC-3'of NS1; Reverse: 5`-CTTCCGTCAATTCCTTTAAG-3` of NS4) and (23SF: 5'-GGACAGA-
AAGACCCTATGAA-3'; 23SR: 5'-TCAGCCTGTTATCCCTAGAG-3') were used for PCR amplification of partial 18S rRNA and 23S rRNA genes respectively [
57,
71]. The primers were prepared by Sangon Biotech Co., Ltd (Shanghai). Each PCR reaction consisted of a 25 µL solution containing 1 µL of genomic DNA, 1 µL of forward primer, 1 µL of reverse primer, 12.5 µL of 2 x PCR TaqMixture (Himedia, India) and 9.5 µL of sterile water. The PCR amplification was performed using following thermal program for 18S rRNA and 23S rRNA amplifications: Preheating at 96 ˚C for 4 min followed by 33 cycles of denaturation at 94 ˚C for 60 s, annealing at 62 ˚C for 60 s, and extension at 72 ˚C for 60 s followed by another 10 min extension at 72 ˚C.
However, the PCR products were sequenced by Sangon Biotech Co., Ltd. (Shanghai, China) and comparison for similar sequences was carried out by BLAST Program (NCBI BLAST, USA). The sequences of the newly isolated algae were deposited into the NCBI GenBank database (National Center for Biotechnology Information, http://www.ncbi.nlm.nih.gov/) [
105] and the accession numbers were obtained (KY656445-KY656464). The isolated strains were identified according to the method described by
Wehr and Sheath [106]. Multiple sequence alignment and phylogenetic tree construction were executed using the Molecular Evolutionary Genetics Analysis v5 (MEGA5) software [
107].
2.5 Wastewater treatment experiment
In order to narrow down the promising isolates in biomass production for lipid accumulation and pollutants removal from wastewaters, all 20 algal isolates were preferably selected conferring to their growth properties, nutrient removals, lipid accumulation self-settleability and algal culture volume index when cultivated in synthetic wastewater that contained MBG11 medium and 10 g L
-1 glucose, for 10 days under dark cultivation (Fig. 2.2). The cultivation of microalgal strains in real domestic wastewater were split into two groups, specifically, using ARDWW and URDWW, so as to check the application scope of these algae considering the symbiotic interactions between microalgae and bacteria. Each culture was conducted in a 250-mL sterilized Erlenmeyer flask with 150 mL URDWW or ARDWW. The dry cell weight (DCW) of microalgae inoculum was around 0.22 g DCW L
-1. The cultivation conditions were under dark condition, pH 7, 150 rpm agitation and 30˚C for 10 days. The mixed liquor was periodically sampled from the flask algae in the course of cultivation. All experiments were conducted in triplicate.
2.6 Adaptation test
The effects of potential microalgal strains in wastewater environment in terms of different temperature, pH and organic sources were investigated after being selected. On behalf of temperature, in order to optimize the promising strains in biomass production and nutrient removal, experiments were carried out at 20, 25, 30, 35 and 40 ˚C temperature levels for carefully chosen strains (due to their performance in RDWW)
Botryococcus sp. NJD-1 and
Scenedesmus sp. NJD-5. Those algae were cultivated under dark cultivation in MBG11 medium supplemented by 10 g L
-1 glucose. The cultivation conditions were pH 7, 150 rpm agitation. Similarly, the performance of microalgal culture at different pH, experiments were done at pH 5, 7, 9 and 11 by the same strains. Growth parameters and nutrient removal rates were determined. The cultivation conditions were 30˚C and 150 rpm agitation. The culture medium used was MBG11 and supplemented by glucose (10 g L
-1) as carbon source.
So far, the adaptation of
Botryococcus sp. NJD-1 strain within numerous carbon types was performed in 10 different kinds of carbon substrates and the growth properties were evaluated. The cultivation conditions were pH 7, 150 rpm agitation and 30˚C and the MBG11 medium complemented with each carbon type was utilized. All experiments were conducted in 250 mL Erlenmeyer flasks with 100 mL culture medium (in triplicate) for 7 days under dark condition using shaker incubator. The dry cell weight (DCW) of microalgae inoculum was around 0.3 g DCW L
-1. The samples were taken periodically after 24 h from the algal flasks in the sequence of the cultivation time.
2.7 Analytical methods
2.7.1 Quality of domestic wastewater used as the culture medium
The concentration of trace metals in domestic wastewater was determined by a multi-channel inductively coupled plasma optical emission spectrophotometer, ICP-OES (PS-4; Baird Co., Bedford, MA, USA). Total organic carbon (TOC) was measured by total organic carbon analyzer (TOC-L CPH, Shimadzu, Japan).
2.7.2 Determination of dry cell weight and evaluation of growth parameters
All 20 microalgal isolates were firstly cultured in heterotrophic conditions and evaluated by measuring the optical density of the sample at absorbance of 685 nm (OD
685 nm) using a Microplate Reader (SpectraMax 190, Molecular devices) after proper dilution with de-ionized water and then selected for other experiments. Each sample among 11 selected candidates was collected during by centrifugation at 10,000 rpm for 10 min with centrifuge (Ankle TGL-16B), and the cell pellets were washed twice with de-ionized water to remove medium salts, dried at 105 ˚С in an oven (DHG-9070A) for 22 h and weighed gravimetrically. The tubes were weighed on a precision analytical balance (Practum 124-1CN, Sartorius) and dry cell weights (DCW) were determined by subtracting the predetermined weight of the empty tube. The initial culture supernatant after the first centrifugation step was carefully removed and used for nutrients analysis. Optical density (OD
685) values was converted into biomass concentration (g L
-1) via appropriate calibration between OD
685 and dry cell weights [
108]. The relationship between OD and DCW was determined through linear regression and converted to (g L
−1). The biomass productivity (
BP, g L
-1 d
-1), the specific growth rate (
μ) and the cell doubling time,
DT in d
-1 (or generation time) were calculated through the Eqs. (2.1) to (2.3), respectively.
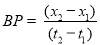
(2.1)
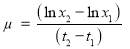
(2.2)
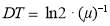
(2.3)
In which
x1 and
x2 are the dry cell weights (g L
−1) on days
t1 (start of the exponential phase) and
t2 (maximum of exponential phase), respectively.
2.7.3 Determination of nutrient concentrations and removal rates
The nitrogen (NO
3-N) concentration in the medium was determined by UV spectrophotometric method. The culture supernatant was collected by centrifugation 10,000 rpm for 10 min by a centrifuge (Ankle TGL-16B). Then the optical density of the sample was measured at a wavelength of 220 nm using a UV/Vis spectrophotometer (UV-1780, Shimadzu, Japan) after proper dilution with deionized water [
97]. The calibration between the absorbance and nitrogen concentration was established using sodium nitrate (NaNO
3) as the standard [
97]. The concentration of phosphorous (PO
4-P) in the medium was determined by ascorbic acid method. Briefly, 0.5 mL supernatant was put in a 10 mL test tube, de-ionized water was added to 5 mL and 0.8 mL color reagent was added. Then, after color development (after 10 min), the absorbance was read at 880 nm using a UV/Vis spectrophotometer (U-1780, Shimadzu, Japan) after proper dilution with de-ionized water. The calibration between the absorbance and phosphorous concentration was established using potassium phosphate monobasic (KH
2PO
4) as the standard in APHA 4500-P [
97]. Total organic carbon (TOC, g L
-1) was measured by total organic carbon analyzer (TOC-L CPH, Shimadzu, Japan). Nutrient removal efficiencies (
RE, %) were calculated using Eq. (2.4). The rate of nutrient removal (
RR, g L
-1 d
-1) was calculated according to Eq. (2.5).
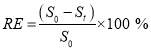
(2.4)

(2.5)
In which
RE is the removal efficiency of substrates (NO
3–N, PO
4–P and TOC);
S0 is the initial concentration and
Stis the maximum concentration of substrates at initial (
to) and final (
tt) time (in days) respectively.
2.7.4 Total lipids extraction by single-step method
Total lipids extraction was performed according to the modification of the method that was previously reported by Axelsson and Gentili [
109]. Freeze-dried biomass (40 mg) by a Freeze dryer (FDU-1200, Eyela) was placed in pre-weighted (
H1) centrifuge tubes, and then 8 mL of chloroform–methanol (2:1 v/v) mixture was added for each strain. Algal biomass was suspended manually by shaking vigorously the centrifuge tubes about 1 min. The mixture was centrifuged at 9,000 rpm and 4 ˚C, for 10 min (Centrifuge 5804 R, Eppendorf). After addition of 2 mL of 0.73 % NaCl aqueous solution to produce a 2:1:0.8 system of chloroform: methanol: water (v/v/v), the mixture was shaken for 20 seconds (Vortex shaker, WH-861) and centrifuged at low speed of 3000 rpm for 2 min to allow phase separation. The upper phase was siphoned and the solvent was evaporated. After the solvent was completely evaporated, lipid fractions were quantified gravimetrically by reweighing (
H2) the centrifuge tubes with a precision analytical balance (Practum 224-1CN, Sartorius).
The percentage of total lipid content (
LC, %) was determined by dividing the weight of recovered lipids by the weight of the dried biomass as Eq. (2.6). All experiments were conducted in triplicate.
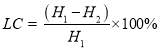
(2.6)
2.7.5 Self-settleability test
After screening all 20 isolates, eleven top growing candidates referring to their optical densities, were tested. The settleability of the microalgae was determined according to the method modified from Manheim and Nelson [
110]. Briefly, it referred to the decrement on the change of OD
685 in 10 mL microalgal suspension distributed in 15 mL centrifuge tubes after 30 min settling. The cultures were gently mixed in 1 min and settled for 30 min at room temperature. An aliquot of the microalgal culture was withdrawn at a height of two-thirds from bottom of the tubes; the OD
685 nm of the cultures at different settling time was measured by UV/Vis spectrophotometer. The settling efficiency (
SE, %) was calculated by Eq. (2.7). All experiments were carried out in triplicate.
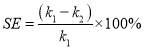
(2.7)
In which
k1 and
k2 are the values of OD
685 nm for the algal suspension at 0 min and 30 min sedimentation time respectively.
2.7.6 Algal culture volume index
The sludge volume index (SVI) is the volume occupied by 1 g of a suspension after 30 min of settling. It is used to monitor settling characteristics of activated sludge and other biological suspensions [
111]. From this, the microalgal cultures were considered. Although SVI is not supported theoretically [
112]. In this particular instance,
ACVI was referred to as algal culture volume index, TSS as microalgal dry cell weight (DCW). In order to calculate the
ACVI, the settled volume (
SV) and the total suspended solids has to be measured. The volume of culture at 30-min of sedimentation was recorded as the settled volume. Algal culture volume index (
ACVI) was calculated as per (Eq. 2.8), also used by Yousuf [
113] with little modifications.
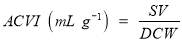
(2.8)
Where
SV is the settled volume in 30 min and
DCW is the dry cell weight after 14-day cultivation of each microalgal candidate.
2.8 Statistical analysis
To determine statistically significant differences between growth condition treatments and strains, OrginPro 8.6 was used to run analysis. Average values of the results of three independent experiments with two analytical replications in each were used.
Chapter 3 Results and Discussion
3.1 Isolation and identification of microalgae from wastewater
A total of 20 heterotrophic microalgal strains were successfully isolated from the local WWTP as summarized in Table 3.1 and 3.2. MBG11 medium isolated higher number of strains than MBold-3N medium. It was observed that all media can support the microalgal growth. An unrooted neighbor-joining phylogenetic tree for the selected 11 strains was reconstructed based on the sequencing results (Fig. 3.1). The morphological analysis and molecular identification of microalgal cultures showed that all the isolates belonged to the genera of
Botryococcus, Chlorella, Scenedesmus, and Nephroselmis. This reflects a diversity of algal strains in domestic wastewater. As shown in Table 3.2, the morphological features of 4 isolates (NJD-2, NJD-5, NJD-6 and NJD-18) that belong to the genus of
Scenedesmus, the family of Scenedesmaceae and the class of Chlorophyceae, were small, non-motile, cylindrical, elongated or fusiform in shape with pointed ends. The colonies were usually observed in groups of 2 or 4 spindle-shaped cells aligned laterally with concave outer side. These observations consist with literature that
Scenedesmus cells are approximately 2-10 µm wide and 5-30 µm long, each cell contains a chloroplast with a pyrenoid and lacks the presence of spines [
114].
Meanwhile, 11 strains among 20 isolates (NJD-3, NJD-7, NJD-8, NJD-9, NJD-10, NJD-11, NJD-12, NJD-13, NJD-14, NJD-15 and NJD-19) were
Chlorella species, i.e., small, non-motile, single-celled green algae, belonging to the family of Chlorellaceae and the class of Trebouxiophyceae. They also conform to the features of
Chlorella species. Specifically, cells were solitary, 2-10 µm in diameter and spherical, globular or ellipsoidal in shape, devoid of flagella, having a parietal and cup-shaped chloroplast (sometimes plate-like) with or without a pyrenoid, and usually overlooked because of their small size. Stemmler et al. [
8] reported that
Scenedesmus and
Chlorella species were mostly isolated species from wastewater treatment effluent, which explains their high occurrence in wastewater samples.
Fig. 3.1 Phylogenetic tree of 11 isolated microalgae and closely related strains from the NCBI nucleotide database. Numbers at the branch nodes are bootstrap values obtained
Table 3.1 Morphology of 20 microalgal strains isolated from WWTP in this study, at 400× magnification
Strain ID |
Microscopic images (400×) |
Strain ID |
Microscopic images (400×) |
Strain ID |
Microscopic images (400×) |
NJD-1 |
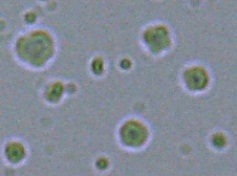 |
NJD-8 |
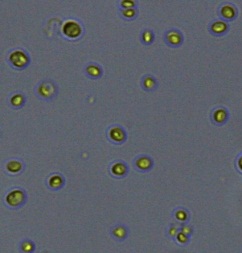 |
NJD-15 |
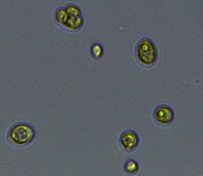 |
NJD-2 |
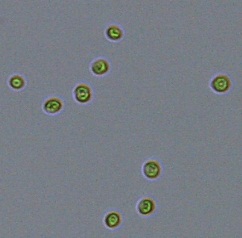 |
NJD-9 |
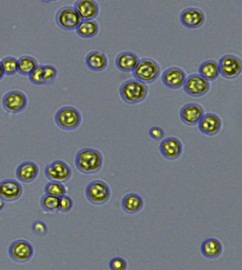 |
NJD-16 |
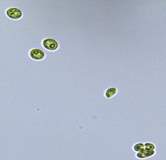 |
NJD-3 |
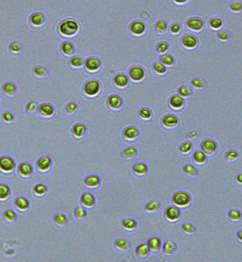 |
NJD-10 |
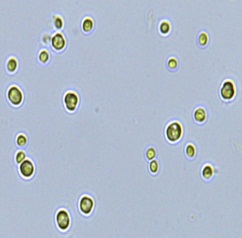 |
NJD-17 |
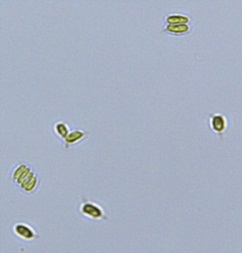 |
NJD-4 |
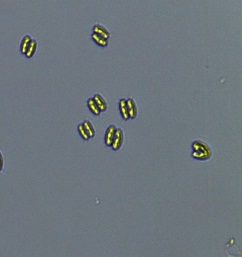 |
NJD-11 |
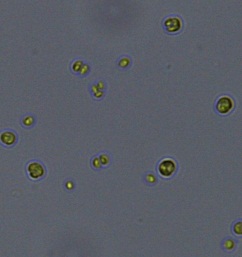 |
NJD-18 |
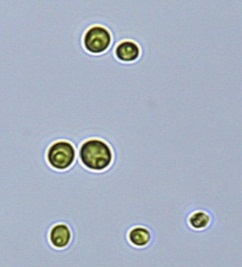 |
NJD-5 |
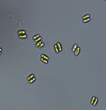 |
NJD-12 |
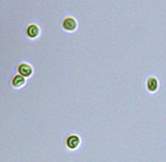 |
NJD-19 |
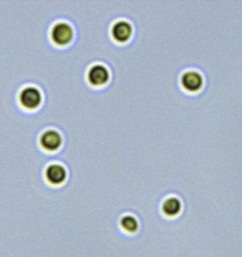 |
NJD-6 |
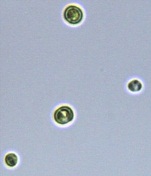 |
NJD-13 |
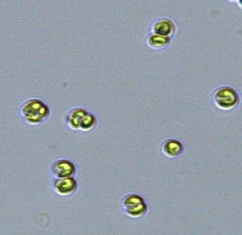 |
NJD-20 |
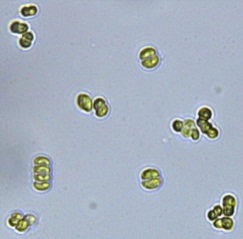 |
NJD-7 |
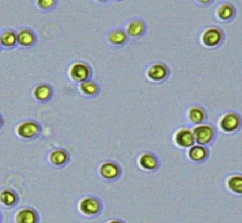 |
NJD-14 |
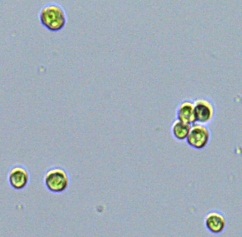 |
|
|
Table 3.2 Molecular identification of 20 microalgae isolated from WWTP in this study
Serial No. |
Microalgal isolates |
Closest BLAST equivalent
and accession number |
Similarity (%) |
Isolating medium |
1 |
Botryococcus sp. NJD-1 |
Botryococcus braunii, KM462884.1 |
84% |
MBG11 |
2 |
Scenedesmus sp. NJD-2 |
Scenedesmus sp. AS-F-7-1, HE717104.1 |
98% |
MBG11 |
3 |
Chlorella sp. NJD-3 |
Chlorella sorokiniana, KJ742376.1 |
100% |
MBold-3N |
4 |
Nephroselmis sp. NJD-4 |
Nephroselmis olivacea, L43358.1 |
82% |
MBG11 |
5 |
Scenedesmus sp. NJD-5 |
Scenedesmus sp. CNW-N, GU939614.1 |
98% |
MBG11 |
6 |
Scenedesmus sp. NJD-6 |
Scenedesmus sp. AS-F-7-1, HE717104.1 |
98% |
MBold-3N |
7 |
Chlorella sp. NJD-7 |
Chlorella variabilis, HQ914635.1 |
98% |
MBG11 |
8 |
Chlorella sp. NJD-8 |
Chlorella vulgaris TMCC6, KP259884.1 |
98% |
MBG11 |
9 |
Chlorella sp.NJD-9 |
Chlorella sorokiniana, KJ397925.1 |
100% |
MBG11 |
10 |
Chlorella sp.NJD-10 |
Chlorella vulgaris ESP-31, GU939612.1 |
99% |
MBold-3N |
11 |
Chlorella sp.NJD-11 |
Chlorella vulgaris ESP-31, GU939612.1 |
99% |
MBG11 |
12 |
Chlorella sp. NJD-12 |
Chlorella sorokiniana, KJ742376.1 |
99% |
MBG11 |
13 |
Chlorella sp. NJD-13 |
Chlorella sp. QUCCCM62, KM985412.1 |
99% |
MBold-3N |
14 |
Chlorella sp. NJD-14 |
Chlorella sorokiniana, KJ397925.1 |
100% |
MBG11 |
15 |
Chlorella sp. NJD-15 |
Chlorella sorokiniana, KJ742376.1 |
100% |
MBG11 |
16 |
Nephroselmis sp.NJD-16 |
Nephroselmis astigmatica, KJ746600.1 |
82% |
MBG11 |
17 |
Nephroselmis sp.NJD-17 |
Nephroselmis olivacea, FN563086.1 |
82% |
BG11 |
18 |
Scenedesmus sp. NJD-18 |
Scenedesmus sp. ESP-5, HE717101.1 |
97% |
MBG11 |
19 |
Chlorella sp. NJD-19 |
Chlorella mirabilis KM462865.1 |
84% |
MBG11 |
20 |
Nephroselmis sp.NJD-20 |
Nephroselmis olivacea, FN563086.1 |
83% |
MBG11 |
Another 4 isolates(NJD-4, NJD-16 NJD-17 and NJD-20) in this study fell in
Nephroselmis genus, the class of Nephrophyceae.
Nephroselmis strains, unicellular freshwater species with contractile vacuole near the flagella bases, have more or less bean-shaped cells with 2 flagella of unequal length. As stated by Calderon and Boo [
115], the cell surface contain the chloroplast with a basal pyrenoid, eyespot and covered with unmineralized scales. Besides, there is another new isolated strain NJD-1, belonging to the genus of
Botryococcus, which is a green microalga classified in Botryococcaceae family (Chlorophyta). NJD-1 strain had 84% homology with the strain
Botryococcus braunii KM462884.1. It is unicellular, planktonic, pyramid-shaped microalga where thin filaments connect the cells which can be 6 to 10 μm long, and 3 to 6 μm wide.
3.2 Preliminary selection in synthetic wastewater
In order to narrow down the promising microalgal strain, among all 20 isolates, 11 highest growing isolates were selected to evaluate their
BP, N-NO
3, P-PO
4, residual TOC concentrations and total lipid content, in the synthetic wastewater. The results in Fig. 3.2 & Table 3.3 showed that the growth of most strains gave the highest cell density within a short time. Under the man-made wastewater environment, the average
BP (g L
-1 d
-1) for
Botryococcus sp. NJD-1,
Chlorella sp. (NJD-7, NJD-9 and NJD-10) were 0.72, 0.61, 0.65 and 0.9; separately at 4-day each. The same maximum biomass yield for
Scenedesmus species (NJD-2, NJD-5 and NJD-6) was obtained as 0.72, 1.19 and 0.48 (g L
-1 d
-1)at 6, 8 and 7-day; respectively, while other
Chlorella sp. (NJD-3 and NJD-8) reached a maximum yield of 0.53 and 0.42 at 6 and 8-day respectively.
Nephroselmis sp. NJD-4 reached 0.7 g L
-1 day
-1 at 8-day (Table 3.3).
Most of isolates show a high drop in organic carbon from 5 to 10 days of cultivation (Fig. 3.2). The highest TOC and NO
3-N removal rates were achieved by
Scenedesmus sp. NJD-2 with 99.2% and 98.4% respectively, but the lowest TOC removal was even 89.7% by
Chlorella sp. NJD-8.
From Fig. 3.2, it was found that most of the strains removed TOC more effectively than the heterotrophic microalgae in literature. For example, the degradation of organic matter with the heterotrophic growth in which COD removal rate of 80.8% was attained by
Chlorella sp. [
116] while
Auxenochlorella protothecoides UMN280 cultivated in MWW reached 92.8% [
9]. Due to their capability to assimilate organic compounds and nutrients, microalgae can play an important role in biological processes associated with bioremediation of wastewaters.
Fig. 3.2 Time-course records of selected microalgal growth curve and nutrients (NO
3-N, PO
4-P and TOC) concentrations in synthetic wastewater
Based on the preferable selection as described in section (2.5), 4 microalgal isolates were further studied for their performance in real wastewater with consideration of the effect of sterilization pretreatment.
Table 3.3 Performance of the 11 selected strains after 10-d cultivation in synthetic wastewater, 30 ˚C, pH 7 and 150 rpm in shaker incubator
Strains
ID |
BP
(gL-1d-1) |
μ
(d-1) |
Lipid yield
(gL-1) |
Lipid content (%) |
|
NJD-1 |
0.72 |
0.77 |
0.53 |
77.4 |
NJD-5 |
1.19 |
0.93 |
0.89 |
75.5 |
NJD-3 |
0.53 |
0.49 |
0.34 |
66.3 |
NJD-2 |
0.72 |
0.51 |
0.41 |
56.6 |
NJD-4 |
0.70 |
0.47 |
0.39 |
55.2 |
NJD-6 |
0.48 |
0.53 |
0.26 |
54.2 |
NJD-11 |
0.42 |
0.44 |
0.21 |
52.3 |
NJD-7 |
0.61 |
0.75 |
0.33 |
51.3 |
NJD-9 |
0.65 |
0.80 |
0.33 |
43.4 |
NJD-10 |
0.90 |
1.03 |
0.33 |
28.8 |
NJD-8 |
0.43 |
0.42 |
0.10 |
23.8 |
3.3 Self-settleability of the isolated microalgae
In this study, the best microalgal strains were selected after analyzing their self-sedimentation and settleability efficiency as summarized in the Fig. 3.3. Among 11 microalgal strains tested for its ability to settle, isolates NJD-8 and NJD-3 showed the highest self-settleability efficiency (after 30 min) of 54.2 and 50.4%, respectively and they were selected as best settling isolates that could be effectively used for microalgal harvesting process. However, most of tested strains showed very low self-settleability efficiency less than 5%. Lananan et al. [
117] reported that some microalgae (e.g.
Chlorella sp.) have densities similar to water which frequently form stable suspensions due to their net negative surface charge. Thus, this makes settling microalgae from wastewater treatment systems difficult. The optical densities of the strains within 60 min were presented (Table 3.4)
Meanwhile, the highest SE producers were not the maximum biomass yielded stains. This phenomenon was also observed by Manheim and Nelson [
110] in which the better settling efficiency of
Chlorella vulgaris during exponential growth attributed to the lower culture density. Higher mixing intensity (150 rpm, in this study) could also improve sedimentation. It was also testified that 100 rpm compared to 75 rpm augmented bioflocculation of
Scenedesmus sp. cultures with
Botryococcus cepacia cell to (85%) [
110].
Table 3.4 Self-sedimentation results for eleven top growing microalgal strains
Strain ID |
Optical density (OD685 nm) values at different time (in minutes) |
1 |
5 |
10 |
15 |
20 |
30 |
40 |
50 |
60 |
NJD-1 |
2.842 |
2.845 |
2.841 |
2.836 |
2.829 |
2.822 |
2.825 |
2.815 |
2.798 |
NJD-5 |
2.971 |
3.002 |
2.951 |
2.942 |
2.913 |
2.852 |
2.867 |
2.664 |
2.518 |
NJD-3 |
2.007 |
1.888 |
1.694 |
1.488 |
1.283 |
1.000 |
0.857 |
0.852 |
0.885 |
NJD-2 |
2.279 |
2.288 |
2.060 |
2.045 |
2.006 |
2.003 |
0.792 |
0.543 |
0.570 |
NJD-4 |
1.893 |
1.886 |
1.878 |
1.867 |
1.848 |
1.850 |
1.706 |
1.629 |
0.536 |
NJD-6 |
1.628 |
1.623 |
1.774 |
1.711 |
1.647 |
1.623 |
1.658 |
1.564 |
1.461 |
NJD-11 |
2.841 |
2.858 |
2.843 |
2.837 |
2.774 |
2.743 |
2.829 |
2.799 |
2.531 |
NJD-7 |
2.947 |
2.881 |
2.853 |
2.841 |
2.838 |
2.816 |
2.355 |
2.208 |
1.995 |
NJD-9 |
1.704 |
1.668 |
1.661 |
1.658 |
1.658 |
1.653 |
1.577 |
1.600 |
1.550 |
NJD-10 |
1.588 |
1.545 |
1.543 |
1.543 |
1.541 |
1.541 |
1.536 |
1.505 |
1.381 |
NJD-8 |
1.746 |
1.404 |
1.193 |
0.957 |
0.961 |
0.796 |
0.702 |
0.505 |
0.502 |
It was also detected that
Scenedesmus species (NJD-2 and NJD-5) at 30-min SE were 12.1 and 2.29% but surprisingly within 60-min, the highest SE reached 74.9 and 71.7 % respectively. The possible reason is the ellipsoid shape and larger size of
Scenedesmus species (2-14 µm wide and 5-30 µm long) used in this study which may have resulted in better settling compared to spherical shape and small species (2-10 µm in diameter and 6-10 µm long). In this regards, it has been witnessed that larger species of
Scenedesmus settled more rapidly than other smaller species [
110].
Generally, NJD-8 strain, due to its highest SE after 30 s, was selected as the energy-efficient algae in terms of harvesting approaches, can offer a cost-competitive production of biofuels and therefore improve concurrent wastewater treatment ability.
3.4 Algal culture volume index (ACVI) of selected microalgal isolates
In this study, for the first time, eleven microalgal strains were compared in terms of settling capacity with the help of
ACVI test, commonly known as SVI (Sludge volume index). Normally, SVI-30 is an important parameter for identifying the settleability properties of sludge.
Fig. 3.3 Microalgal biomass concentration and their respective settleability efficiencies cultivated in synthetic medium
In this particular study, SVI-30 referred to as algal culture volume index (
ACVI-30) where isolated microalgae interact with the synthetic medium (MBG11). From Fig. 3.3,
ACVI data and their respective dry cell weights.
ACVI was calculated through (Eq. (2.7)) within 30 minutes and it was directly affected by dry biomass. Due to the lack of literature, results of microalgal settling are here compared with flocculated waste activated sludge. According to Yousuf [
113],
ACVI-30 range can be in 3 groups. When
ACVI is less than 100 mL g
-1 attributed to old sludge or microalgal suspension, possible flocs and increasing turbidity, hence high settling efficiency will be observed. In this range there were no observed strains.
When
ACVI ranged between 100-250 mL g
-1, strains show good settling with normal operation and low effluent turbidity [
113]. Microalgal culture specifically
Chlorella sp. NJD-8 (113.9 mL g
-1),
Nephroselmis sp. NJD-4 (166.7 mL g
-1),
Scenedesmus sp. NJD-2 (211.1 mL g
-1) and
Scenedesmus sp. NJD-5 (181.6 mL g
-1) were found to be in this range while the remaining strains, their
ACVI were greater than 250 mL g
-1 which were previously reported to show poor settling and burking sludge (or microalgal suspension) confirming high effluent turbidity [
113]. It was observed that
ACVI decreased as well as the biomass concentration increases (Fig. 3.3).
For example NJD-4, NJD-2 and NJD-5 strains have 5.7, 4.9 and 4.5 g DCW L
-1, respectively. They were considered as the highest biomass producers compared to others in this study. On the other hands,
Chlorella species (NJD-13 and NJD-7 mL g
-1) with the lowest dry biomass of 1.2 and 2.6 g L
-1 have the largest
ACVI of 837.6 and 361.5 mL g
-1 respectively, which demonstrate poor settleability. Anbalagan et al. [
118] showed that microalgae grown photosynthetically can vary in size from 1 to 50 mm depending on the strain (e.g.
Chlorella 1-8 mm), which is larger compared to the size of activated sludge bacteria (0.2-5 mm) and microalgae reproduce slowly and form floating flocs together with bacteria in the wastewater to increase their population density [
119]. A higher SVI correlates with poorer settleability of the sludge. Sludge with a SVI of more than 150 mL g
-1 is considered to have poor settleability [
118]. Furthermore, in order to select the best strains for RDWW among eleven used microalgae species, according to
ACVI test, four promising, cost-effective isolates which had good prospects for further utilization namely
Scenedesmus sp. NJD-2,
Nephroselmis sp. NJD-4,
Scenedesmus sp. NJD-5 and
Chlorella sp. NJD-8 were selected.
3.5 Performance of the isolated microalgae in real wastewater
In this study, it was observed that some microalgae strains failed to grow in the real wastewater (data not shown), reaching the decline phase within 2 days and the cells even disrupted after 3 days. The main reason for poor microalgal growth in the real wastewater might be the inevitable contamination of bacteria which could be observed by the light microscope. Wang et al. [
120] indicated that the cultivation of microalgae within real wastewater is a complicated symbiotic system where the contamination by biological pollutants is inevitable. This can become a big constraint in mass cultivation and hinder the large-scale process.
Table 3.5 Biomass yield and lipid production of new isolated strains in wastewaters compared to chlorophyta species previously studied under heterotrophic cultivation
Serial No. |
Microalgae |
Wastewater used |
Biomass yield (g L-1) |
Lipid content (%) |
Ref. |
1 |
Botryococcus sp. NJD-1
Scenedesmus sp. NJD-2
Scenedesmus sp. NJD-5
Chlorella sp. NJD-3 |
URDWW |
1.17
2.02
0.54
1.61 |
61.7
50.4
55.9
40.1 |
This study |
|
Botryococcus sp. NJD-1
Scenedesmus sp. NJD-2
Scenedesmus sp. NJD-5
Chlorella sp. NJD-3 |
ARDWW |
0.93
1.19
0.43
0.76 |
55.8
43.3
50.2
34.8 |
|
2 |
Scenedesmus sp. ZTY2,
Scenedesmus sp. ZTY3
Chlorella sp. ZTY4 |
ARDWW |
1.65
1.98
2.31 |
69.1
55.3
79.2 |
[7] |
3 |
Chlorococcum sp. RAP13 |
ADE |
1.94 |
42 |
[69] |
4 |
Scenedesmus sp. LX1 |
ARDWW |
0.11 |
31–33 |
[121] |
5 |
Auxenochlorella
Protothecoides UMN 280 |
AMWW |
1.12 |
28.9 |
[9] |
6 |
Mixed culture |
URDWW |
3.2 |
27.8 |
[122] |
7 |
Chlorella sp. UM224
Scenedesmus sp. UM284
Hindakia sp. UM265 |
ACMWW |
1.4
1.5
1.7 |
33.5
30.1
28.3 |
[28] |
8 |
Scenedesmus sp. |
Fructose, glucose and acetate |
3.46 |
52.6 |
[123] |
Hence, 4 newly isolated microalgal strains namely
Botryococcus sp. NJD-1,
Scenedesmus sp. NJD-2,
Chlorella sp. NJD-3 and
Scenedesmus sp. NJD-5 able to grow in real wastewater were studied with ARDWW and URDWW comparatively (Figs. 3.4 and 3.5). As shown in Table 3.5, the maximum biomass yield (g DCW L
-1) of NJD-1, NJD-2, NJD-3 and NJD-5 with ARDWW were 0.93 ± 0.071, 1.19 ± 0.039, 0.43 ± 0.009 and 0.76 ± 0.059, respectively. The algal strains showed exponential growth during the first 3-7 days without any lag phase indicating their adaptability of to the local wastewater surroundings (Fig. 3.4). Contrary, the maximum biomass yield (g DCW L
-1) for the same strains cultured in URDWW were 1.17 ± 0.002, 2.02 ± 0.027, 0.54 ± 0.025 and 1.61 ± 0.007, separately.
It reflects that the selected microalgae successfully grew in RDWW under heterotrophic conditions. These algal strains showed their great potential for local wastewater treatment, suggesting that these wastewater cultures are capable to support microalgal growth necessities. This agreed with Zhou et al. [
28] that the isolated algae from real wastewater systems can grow well and frequently adjust to growth conditions comparable to where they are originated.
It was found that the microalgal growth was higher under URDWW cultivation phase compared to grown cells in ARDWW medium. Obviously, this was expected by means of the URDWW would have contained initial microalgal concentrations. These results also corresponded with previous reports in which autoclaved domestic wastewater [
7,
28], DE [
69] and MWW [
9] were used as media to culture several microalgal species and also produced high biomass growth rates and bio-energy.
Likewise, 4 selected microalgae cultured to treat RDWW for 10 days, showed good ability to accumulate high lipid content (Table 3.5).
Botryococcus sp. NJD-1 was the best for lipid accumulation in our study (61.7%), while lipid content of the other three was in the range of 34.8-55.9%. These values were higher than most lipid contents of the algae cultivated within wastewaters. For instance, culturing
Chlorococcum sp. RAP13 in autoclaved dairy effluent, 42% DCW was achieved [
69],
Scenedesmus sp. LX1 used to treat autoclaved RDWW reached (31-33%) [
121] and only running after one data obtained by
Chlorella sp. ZTY4 using sterile wastewater, 79.2% DCW was reached [
7], while mixed culture in untreated RDWW obtained only 27% [
122]. These results thereby indicate the potential of the tested isolates NJD-1, NJD-2, NJD-3 and NJD-5 as prospective sources of biofuel in practice of pristine wastewater cultivation. They showed good ability to accumulate lipid.
The pollutants removal results of the selected microalgae in ARDWW and URDWW were summarized in Table 3.6, with comparison of previous studies. It showed that the NO
3-N removal efficiencies were slightly higher in URDWW (50.1-64.5%) than in ARDWW (37.1-46.3%) for NJD-1, NJD-2, NJD-3 and NJD-5 strains.
This is undoubtedly due to ubiquitous naturally occurring microalgae in URDWW which contribute to the uptake of nitrate nitrogen whereas autoclaving devastated any of the naturally arising microalgae as well as any bacteria. The NO
3-N declined from initial concentrations of 15.4 to 8.26 mg L
-1 (46.3%) for
Scenedesmus sp. NJD-2 in ARDWW and from 15.8 to 5.59 mg L
-1 (64.5%) for
Botryoccocus sp. NJD-1 in URDWW (Fig. S4 and S5), proposing that NJD-1 and NJD-2 showed high nitrogen concentration tolerance during real wastewater treatment.
Fig. 3.4 Time-course records (curves) of (a) biomass concentration, (b) nitrogen concentration, (c) phosphorus concentration and (d) TOC concentration for algal strains NJD-1, NJD-2, NJD-5 and NJD-3 cultivated in ARDWW
The fact that only (37.1-64.5%) of total nitrogen was removed in both wastewater media, in this study, indicating that in the culture solutions, there were still some nitrogen compounds such as NH
4-N, urea, acetic acid, amino acids, etc. that could not be converted to nitrate nitrogen or assimilated by microalgae. From results, it is remarkable that the nitrogen removal was much greater than those reported using RDWW [
7,
124]. In previous studies, Zhou et al. [
9] cultivated
A. protothecoides UMN280 to treat MWW where total nitrogen (TN) was drastically reduced to 59.7% and
Chlorella sp. ZTY4 formerly used to remediate DWW reached 15.8% TN drop [
7].
Phosphorus (PO
4-P) removal pattern was much higher compared to nitrate nitrogen deduction in 4 microalgae tested. In URDWW culture medium, PO
4-P removal rate was considerably dropped from 2.65-2.67 to 0.27-0.61 mg L
-1 for
Botryococcus sp. NJD-1,
Scenedesmus sp. NJD-2,
Chlorella sp. NJD-3 and
Scenedesmus sp. NJD-5 where NJD-1 reached the highest
RE (89.8%) during the first 4 days of cultivation. It was found that maximal removal efficiency was ranged from 77.4 to 89.8% and this result was so much higher than that reported by Prathima Devi et al. [
124], where mixed microalgae in URDWW obtained only 32.8%. The removal efficiencies of all microalgae showed no big considerable differences.
Similarly, when the same strains were cultured in ARDWW, the lowest PO
4-P removal efficiency was observed on
Chlorella sp. NJD-3 (79.1%) while the highest
RE was detected on
Scenedesmus sp. NJD-5 (96.1%). The concentration of PO
4-P lessened dramatically from 2.57 to 0.10-0.54 mg L
-1 within the first 3-4 days of heterotrophic cultivation (Fig. 3.4). It is clear that the results obtained in both wastewater cultures have achieved much higher PO
4-P removal efficiencies compared to preceding reports using different wastewaters. For example, 3 species (
Scenedesmus sp. ZTY2,
Scenedesmus sp. ZTY3 and
Chlorella sp. ZTY4) in ARDWW gained (32.7-49.9%) [
7],
Chlorella sp. cultured in wastewater from sludge centrifuge reached 85.6 % [
125], mixed microalgae in domestic wastewater obtained only 32.8% [
122] while
A. protothecoides UMN280 reached 81.5% [
9] when cultivated in AMWW. Phosphorus removal efficiency over 87% for almost all strains was very effective. This high removal efficiency indicates high phosphorus concentration tolerance of naturally wastewater-born microalgae.
Fig. 3.5 Time-course records (curves) of (a) biomass concentration, (b) nitrogen concentration, (c) phosphorus concentration and (d) TOC concentration for algal strains NJD-1, NJD-2, NJD-5 and NJD-3 cultivated in URDWW
So far, TOC removal in ARDWW was also studied and presented (Table 3.6 and Fig. 3.4). The TOC removal efficiencies of NJD-3 and NJD-5 were almost complete (> 99%) within 5 and 8 cultivation days respectively. Those results are much higher compared with the literature in which
A. protothecoides UMN280 extended to 92.8% [
9] in MWW medium. And NJD-1 and NJD-2 dropped (mg L
-1) from 77.97-19.12 (75.5%) and 78.1-9.01 (88.5%) within 4 and 7 days respectively. These data are also comparable with earlier study.
Table 3.6 Comparison on performance of microalgae to treat nutrients from RDWW and reported wastewater systems under heterotrophic cultivation
Microalgal
strains |
Type of WW |
N concentration |
P concentration |
TOC |
Refs. |
Initial
(mg L−1) |
RE
(%) |
Initial
(mgL−1) |
RE
(%) |
Initial
(mgL−1) |
RE
(%) |
Botryococcus sp. NJD-1 |
URDWW |
15.8 NO3-N |
64.5 |
2.65
PO4-P |
89.8 |
79.0 |
67.9 |
This study |
Scenedesmus sp. NJD-2 |
15.8 NO3-N |
52.2 |
2.67
PO4-P |
88.5 |
79.1 |
70.8 |
Chlorella sp. NJD-3 |
15.8 NO3-N |
50.1 |
2.67
PO4-P |
77.4 |
79.0 |
65.1 |
Scenedesmus sp. NJD-5 |
15.7 NO3-N |
51.1 |
2.65
PO4-P |
87.7 |
79.2 |
73.9 |
Botryococcus sp. NJD-1 |
ARDWW |
15.5 NO3-N |
41.1 |
2.57
PO4-P |
89.5 |
77.9 |
75.5 |
Scenedesmus sp. NJD-2 |
15.4 NO3-N |
46.3 |
2.57
PO4-P |
87.8 |
78.1 |
88.5 |
Chlorella sp. NJD-3 |
15.4 NO3-N |
37.1 |
2.57
PO4-P |
79.1 |
77.0 |
99.1 |
Scenedesmus sp. NJD-5 |
15.4 NO3-N |
45.5 |
2.57
PO4-P |
96.1 |
78.1 |
99.9 |
Scenedesmus sp. ZTY2 |
ARDWW |
27.7 TN |
14 |
1.59 TP |
35 |
|
|
[7] |
Scenedesmus sp. ZTY3 |
27.7 TN |
9.8 |
1.59 TP |
32.7 |
|
|
|
Chlorella sp. ZTY4 |
27.7 TN |
15.8 |
1.59 TP |
49.9 |
|
|
|
Chlorella sp. |
ACMWW
UCMWW |
132.3 TN
116.1 TN |
89.9
89.1 |
215 PO4-P
212 PO4-P |
79.0
80.9 |
2390
2304 |
90.3
90.8 |
[81] |
Chlorella sp. |
WW from sludge |
131.1 TN
71.8
NH3-N |
82.8
78.3 |
201.5
PO4-P |
85.6 |
|
|
[125] |
Chlorella sorokiniana
UTEX 1670 |
Proteose medium
+ glucose |
119.8 NO3-N |
48 |
33.3
PO4-P |
40 |
|
|
[126] |
Mixed microalgae |
URDWW |
115
NO3-N |
36.8 |
48
PO4-P |
32.8 |
|
|
[124] |
A.protothecoides UMN280 |
AMWW |
134 TN
91 NH3-N |
59.7
69 |
211
PO4-P |
81.5 |
970 |
92.8 |
[9] |
In Shen et al. [
127], the average TOC removal efficiency of
Scenedesmus obliquus in effluent from a wastewater treatment workshop was 85.3%. Other studies [
128] also revealed that the nutrient removal efficiency is related to the level of nutrients in wastewater and the extent of nutrients utilized by algal growth or incorporated into algal tissues. In URDWW, percentage removal efficacy of TOC was 67.9%, 70.8%, 65.1% and 73.9% for NJD-1, NJD-2, NJD-3 andNJD-5 respectively (Table 3.6 & Fig. 3.5). It is flawless that autoclaved pilot reached higher TOC removal efficiencies than untreated phase. The probable reason was the reduction of nutrients during autoclaving in which the complex organic substrates were probably broken down to simple molecules easy to be assimilable by microalgae.
From the literature, Ramsundar et al. [
70] reported that autoclaving might have an effect on microalgal growth as it reduces nutrients from wastewater so that chemical nutrients can be supplemented to enhance the cell growth, but this can also be impractical at large scale. It is worth declared that, while the removal of the nutrients was significant, the concentration remaining in the domestic wastewater was still high especially for nitrogen. In order to encounter the wastewater discharge criteria, additional nutrients removal processes are required.
3.6 Adaptation of isolated microalgal strains in wastewater environment
3.6.1 Effect of temperature on algal performance
Temperature as environmental factor can play a significant stress role in algal microalgal growth, physiology, morphology, lipid production and nutrient supplies [
129]. The experiments were operated at 20, 25, 30, 35 and 40 ˚C temperatures for two strains able to effectively perform in RDWW (
Botryococcus sp. NJD-1 and
Scenedesmus sp. NJD-5), in order to investigate their optimum cultivation temperatures. According to the results shown in Fig. 3.6, it is remarkable that the microalgal growth of NJD-1 increased from 20 to 30 ˚C and then gradually decreased from 35 to 40 ˚C. Generally, this species grew well at all scenarios tested (20-40 ˚C) producing quite similar biomass yield. Specifically, it shows the high growth rate at 40 ˚C, indicating its tolerance at high temperature. Similarly for NJD-5 stains, the cell growth steadily increased from 20 to 30 ˚C and at that point decreased to 40 ˚C.
It is seen that the growth rate at 20 ˚C and 40 ˚C were weak and decayed slowly after 3 days. Maximum cell density was noticed at 30 ˚C (4.1 ± 0.06 and 5.1 ± 0.04 g L
-1) for NJD-1 and NJD-5, individually. From the literature, the same events were observed in which microalgal growth rate were improved with the increase in temperature up to its optimum and growth rate decreased as soon as it reaches its optimum [
129,
130]. Also, the increase in biomass yield was reported at optimum temperature of 30 ˚C [
130,
131]. The minimum biomass concentrations were recorded at 20 ˚C and 40 ˚C for NJD-1 and NJD-5 respectively whereas the maximum one was found at 30 ˚C for both strains. This might be due to the lessened photosynthetic activity prominent to enzyme instability [
130]. Temperature also plays a key role in photo-inhibition, which is known to impact algal growth rate.

DCW for NJD-1

DCW for NJD-5
Fig. 3.6 Effect of temperature on microalgal strains: nutrient removal efficiency (NO
3-N and PO
4-P) and dry biomass concentration at different temperature
Concerning the nutrient removal (Fig. 3.6), NJD-1 reached 97.1 ± 0.79% and 95.3 ± 0.05% for N-NO
3 and P-PO
4 at 25 and 30 ˚C separately whereas
Scenedesmus sp. NJD-5 grasped 98.4 ± 0.78% nitrate nitrogen reduction and 96.0 ± 0.06% PO
4-P of RE at 30 ˚C, individually. This phenomena was coincided with the study of Subhash et al. [
130] which has shown that as microalgal cells increasing gradually from lower temperature to the optimum, it is clear that the nutrient concentrations in the culture also will be gradually up-taken to the maximum removal rates (at optimum), and then slightly increase as the cell growths decreased.
Generally, both
Botryococcus sp. NJD-1 and
Scenedesmus sp. NJD-5 were detected to grow better at 30 ˚C, and specifically NJD-1 can tolerate to high temperature. Temperature being an important factor for growing algae strongly influences the growth rates, the cellular lipid composition, the uptake of nutrients and carbon fixation.
3.6.2 Effect of pH on algal performance
The growth of microalgae is openly affected by the availability of nutrients, pH, temperature, light and the initial inoculum’s density [
85]. Each microalga has its own optimal growth pH. Fig. 3.7 show the results of
Botryococcus sp. NJD-1 and
Scenedesmus sp. NJD-5 from experiments operated at 5, 7, 9 and 11 pH levels. The suitable pH for NJD-1 and NJD-5 was observed at pH 7; at which the maximum biomass productivity were 1.24 g L
-1 d
-1 and 1.87 g L
-1 d
-1 respectively. The highest nitrogen and phosphorus removal efficiencies were obtained at pH 7 while the lowest growth and removal rate were found at pH 5.
For the experiment studying the nutrient removal of selected two species
Botryococcus sp. NJD-1 and
Scenedesmus sp. NJD-5 at different pH levels from synthetic wastewater, the results were presented in Fig. 3.7. For instance, the monoculture of
Botryococcus sp. NJD-1 had the highest daily nitrogen removal rate (maximally 27.6 mg NO
3-N L
-1 d
-1) in the synthetic wastewater at pH 7), while the monoculture of
Scenedesmus sp. NJD-5 had the highest nitrogen removal rate (maximally 15.5 mg NO
3-N L
-1 d
-1 at pH 7). In terms of phosphorus removal, the monoculture of
Botryococcus sp. NJD-1 had the highest daily phosphorus removal rate (maximally 6.8 mg PO
4-P L
-1 d
-1) in the synthetic wastewater, while
Scenedesmus sp. NJD-5 reached 15.9 mg NO
3-N L
-1 and 7.3 mg PO
4-P L
-1 d
-1 removal rates.
Fig. 3.7 Nutrients removal profiles: (a) and (c) for
Botryococcus sp. NJD-1; (b) and (d) for
Scenedesmus sp. NJD-5 cultivated for 7 days at different pH heterotrophically
After 7 days cultivation, the nitrogen removal efficiency of
Scenedesmus sp. NJD-5 (31.7 - 94.4%) was slightly higher than
Botryococcus sp. NJD-1 (66.3 - 94.2 %) for the four different pH scenarios (Fig. 3.7). In other hands, the phosphorus removal efficiency of
Botryococcus sp. NJD-1 (62.4 - 94.1%) was slightly higher than
Scenedesmus sp. NJD-5 (65.7 - 90.0 %) for the four different pH settings.
The difference in the maximal phosphorus removal rate for two strains in wastewater could probably be caused by the chemical precipitation of phosphorus. Chemical precipitation has been documented as an important phosphorus removal mechanism from the wastewater by algae [
132]. In addition, The increase of pH in cultures could help contribute to the precipitation of phosphorus and the increase of phosphate adsorption on microalgal cells [
133] and thus the decrease of phosphorus levels in cultures occurred suggesting that the algae species used in this study is high phosphorus concentration tolerance. The pH of the wastewater can be prompted by the consumption of CO
2 through the algal photosynthesis and then result in the phosphorus precipitation [
134-136]. Moreover, phosphorus can also be removed through surface adsorption by benthic algal community via the formation of hydroxyapatite [
137,
138]. Accordingly, because of the diverse phosphorus removal processes, the effect of flow rate on phosphorus removal was inhibited.
3.6.3 Effect of organic type on algal performance
Due to the high cost of artificial mediums, commercial application of bioenergy based on heterotrophic microalgal cultivation is limited and even glucose can extent to about 80% of the total cost of the medium [
20]. The studies indicated that besides glucose and acetate, microalgal strains possibly could assimilate numerous other kinds of organic substrates. Tian-Yuan et al. [
67] evaluated the heterotrophic cultivation of microalgae in 31 substrates using the Biolog-ECO Microplate.
Table 3.7 Effects of different carbon sources on the growthof
Botryococcus sp. NJD-1
Categories of Carbon source |
Carbon substrate |
DCW
(g L-1) |
BP
(g L-1 day-1) |
µ
(day-1) |
DT
(d-1) |
Carbohydrates |
Na acetate |
2.9 ± 0.01 |
0.9 ± 0.004 |
1.1 ± 0.020 |
0.6 ± 0.01 |
Xylose |
1.5 ± 0.01 |
0.2 ± 0.000 |
0.3 ± 0.003 |
2.7 ± 0.03 |
α-Lactose |
1.6 ± 0.01 |
0.2 ± 0.001 |
0.3 ± 0.001 |
2.5 ± 0.01 |
Glycerol |
1.0 ± 0.02 |
0.2 ± 0.013 |
0.4 ± 0.014 |
1.9 ± 0.08 |
D-Galactose |
3.0 ± 0.00 |
0.4 ± 0.001 |
0.4 ± 0.002 |
2.0 ± 0.01 |
Carboxylic acids |
Pyruvic acid |
1.0 ± 0.01 |
0.01± 0.003 |
0.03 ± 0.00 |
27 ± 0.03 |
DL-Malic acid |
- |
- |
- |
- |
Maleic acid |
- |
- |
- |
- |
Amino acids |
Glutamic acid |
0.1 ± 0.01 |
0.004 ± 0.0 |
0.02 ± 0.0 |
35 ± 0.02 |
Polymers |
Starch soluble |
1.4 ± 0.02 |
0.3 ± 0.001 |
0.003 ± 0.0 |
1.5 ± 0.01 |
In this study, 10 organic substrates classified in four categories; carbohydrates, carboxylic acids, amino acids and polymers were used to grow
Botryococcus sp. NJD-1. Based on the result, 7 kinds of substrates can be metabolized (Table 3.7). Thus, this strain was considered to obtain the strong heterotrophic growth capacity which shows the possibility to treat different types of wastewaters.
Fig. 3.8 Growth curve of
Botryococcus sp. NJD-1 cultivated in different carbon sources for 7 days under dark conditions
The growth parameters of
Botryococcus sp. NJD-1 for each carbon substrate under dark conditions were presented in the Table 3.7. In this report, the top three assimilable substrates with their biomass productivity (g L
-1 day
-1) were Na acetate (0.9 ± 0.004), D-Galactose (0.4 ± 0.001) and Starch soluble (0.3 ± 0.001) while in DL-Malic acid and Maleic anhydride there was no significant growth, which indicates that the substrates can hardly be metabolized.
Due to the high cost of artificial mediums, commercial application of bioenergy based on heterotrophic microalgal cultivation is limited. The studies indicated that besides glucose and acetate, microalgal strains possibly could assimilate several other kinds of organic substrates. Zhang et al. [
139] evaluated the heterotrophic cultivation of microalgae in 31 substrates using the Biolog-ECO Microplate, but the growth features were not assessed. In this study, 10 organic substrates classified in four categories; carbohydrates, carboxylic acids, amino acids and polymers were used to grow
Botryococcus sp. NJD-1. The growth parameters of
Botryococcus sp. NJD-1 for each carbon substrate under dark conditions were presented (Table 3.7).
Due to the high cost of artificial mediums, commercial application of bioenergy based on heterotrophic microalgal cultivation is limited. The studies indicated that besides glucose and acetate, microalgal strains possibly could assimilate several other kinds of organic substrates. Zhang et al. [
139] evaluated the heterotrophic cultivation of microalgae in 31 substrates using the Biolog-ECO Microplate, but the growth features were not assessed. In this study, 10 organic substrates classified in four categories; carbohydrates, carboxylic acids, amino acids and polymers were used to grow
Botryococcus sp. NJD-1. The growth parameters of
Botryococcus sp. NJD-1 for each carbon substrate under dark conditions were presented (Table 3.7).
In this study, the top three assimilable substrates with their biomass productivity (g L
-1 day
-1) were sodium acetate (0.9 ± 0.004), D-Galactose (0.4 ± 0.001) and starch soluble (0.3 ± 0.001) while in DL-Malic acid and Maleic anhydride there was no significant growth. Growth curve of
Botryococcus sp. NJD-1 in different carbon sources (Fig. 3.8) showed that 7 kinds of substrates could be metabolized, suggesting that the microalgae used in this study could rapidly utilize different organic compounds as carbon sources besides CO
2. Also, this strain showed the strong heterotrophic growth capacity which displays the possibility to treat different types of wastewaters. Previously, Wu and Wang [
20] developed a co-culture system by mixing
C. pyrenoidosa and
R. glutinis using sucrose as the sole carbon source wherein the highest cell density of 111.48 × 10
6 cells mL
-1 was obtained. Additionally, it is reported that manymicroalgal species can grow heterotrophically, mixotrophically and autotrophically on multiplicity of organic carbon [
19,
81], suggesting that they can simultaneously use light and organic source as energy sources and assimilate CO
2 and glucose or simple acids as carbon sources.
In this study, the results showed that all carbohydrates substrates were easily metabolized compared to others organic carbon categories followed by starch soluble whereas carboxylic acids and amino acids substrates were hardly assimilable by
Botryococcus sp. NJD-1. This analysis could be used to determine which category of substrates suitable for specific wastewater treatment by heterotrophic microalgal strains.
Chapter 4 Conclusions
Microalgal growth is the key to the coupled system of wastewater treatment and algal biomass-based lipid production. Total of 20 native heterotrophic microalgal strains were isolated from local domestic wastewater, identified and selected for concurrent pollutants removal from wastewater and biofuel production heterotrophically.
After the selection through a comprehensive criterion involving lipid accumulation, pollutants removal, self-settleability and algal culture volume index, four strains (
Botryococcus sp. NJD-1,
Scenedesmus sp. NJD-2,
Chlorella sp. NJD-3 and
Scenedesmus sp. NJD-5) were applied back to the original URDWW, achieving excellent effect even superior to ARDWW. Especially, NJD-1 exhibited a strong capacity to simultaneously accumulate lipid up to 61.7% and remove N/P/TOC above 64.5% in URDWW, as well as assimilating a wide range of carbon substrates and NJD-1 could tolerate to high temperature (40˚C). The suitable pH for growth and nutrient removal of NJD-1 and NJD-5 was at pH 7; at which biomass productivity were 1.3 and 1.9 g L
-1 d
-1 respectively, while the lowest growth and removal rate were found at pH 5 for both strains.
Reference
[1] E. Tilley, L. Ulrich, C. Lüthi, P. Reymond, C. Zurbrügg, Compendium of sanitation systems and technologies. Swiss Federal Institute of Aquatic Science and Technology (Eawag), Duebendorf, Switzerland, 2008. ISBN 978-3-906484-57-0.
[2] J. Liu, Z. Sun, H. Gerken, Potential applications of microalgae in wastewater treatments, Recent Advances in Microalgal Biotechnology; OMICS Group e-Books: Hyderabad, India, (2014).
[3] A.K. Kivaisi, The potential for constructed wetlands for wastewater treatment and reuse in developing countries: a review, Ecological Engineering, 16 (2001) 545-560.
[4] R.S. Ramalho, Introduction to wastewater treatment processes (Second Edition), ISBN: 9780080925332, Academic Press, San Diego (1983) 1-571.
[5] O. Zmora, A. Richmond, 20 microalgae for aquaculture. Handbook of microalgal culture: Biotechnology and Applied Phycology, (2004) 365.
[6] Y. Li, W. Zhou, B. Hu, M. Min, P. Chen, R.R. Ruan, Integration of algae cultivation as biodiesel production feedstock with municipal wastewater treatment: strains screening and significance evaluation of environmental factors, Bioresource Technology, 102 (2011) 10861-10867.
[7] T.-Y. Zhang, Y.-H. Wu, S.-f. Zhu, F.-M. Li, H.-Y. Hu, Isolation and heterotrophic cultivation of mixotrophic microalgae strains for domestic wastewater treatment and lipid production under dark condition, Bioresource Technology, 149 (2013) 586-589.
[8] K. Stemmler, R. Massimi, A.E. Kirkwood, Growth and fatty acid characterization of microalgae isolated from municipal waste-treatment systems and the potential role of algal-associated bacteria in feedstock production, PeerJ, 4 (2016) e1780.
[9] W. Zhou, M. Min, Y. Li, B. Hu, X. Ma, Y. Cheng, Y. Liu, P. Chen, R. Ruan, A hetero-photoautotrophic two-stage cultivation process to improve wastewater nutrient removal and enhance algal lipid accumulation, Bioresource Technology, 110 (2012) 448-455.
[10] L. Li, J. Cui, Q. Liu, Y. Ding, J. Liu, Screening and phylogenetic analysis of lipid-rich microalgae, Algal Research, 11 (2015) 381-386.
[11] A. Jebali, F.G. Acién, C. Gómez, J.M. Fernández-Sevilla, N. Mhiri, F. Karray, A. Dhouib, E. Molina-Grima, S. Sayadi, Selection of native Tunisian microalgae for simultaneous wastewater treatment and biofuel production, Bioresource Technology, 198 (2015) 424-430.
[12] I.T.D. Cabanelas, M.v.d. Zwart, D.M.M. Kleinegris, M.J. Barbosa, R.H. Wijffels, Rapid method to screen and sort lipid accumulating microalgae, Bioresource Technology, 184 (2015) 47-52.
[13] F. Roselet, D. Vandamme, M. Roselet, K. Muylaert, P.C. Abreu, Screening of commercial natural and synthetic cationic polymers for flocculation of freshwater and marine microalgae and effects of molecular weight and charge density, Algal Research, 10 (2015) 183-188.
[14] E.B. Sydney, T.E. da Silva, A. Tokarski, A.C. Novak, J.C. de Carvalho, A.L. Woiciecohwski, C. Larroche, C.R. Soccol, Screening of microalgae with potential for biodiesel production and nutrient removal from treated domestic sewage, Applied Energy, 88 (2011) 3291-3294.
[15] J.-C. Frigon, F. Matteau-Lebrun, R. Hamani Abdou, P.J. McGinn, S.J.B. O’Leary, S.R. Guiot, Screening microalgae strains for their productivity in methane following anaerobic digestion, Applied Energy, 108 (2013) 100-107.
[16] Z. Tian-Yuan, W. Yin-Hu, Z. Lin-Lan, W. Xiao-Xiong, H. Hong-Ying, Screening heterotrophic microalgal strains by using the Biolog method for biofuel production from organic wastewater, Algal Research, 6, Part B (2014) 175-179.
[17] Z. Sun, Z.-g. Zhou, H. Gerken, F. Chen, J. Liu, Screening and characterization of oleaginous
Chlorella strains and exploration of photoautotrophic
Chlorella protothecoides for oil production, Bioresource Technology, 184 (2015) 53-62.
[18] Y.-H. Wu, X. Li, Y. Yu, H.-Y. Hu, T.-Y. Zhang, F.-M. Li, An integrated microalgal growth model and its application to optimize the biomass production of
Scenedesmus sp. LX1 in open pond under the nutrient level of domestic secondary effluent, Bioresource Technology, 144 (2013) 445-451.
[19] J.-M. Girard, R. Tremblay, N. Faucheux, M. Heitz, J.-S. Deschênes, Phycoremediation of cheese whey permeate using directed commensalism between
Scenedesmus obliquus and
Chlorella protothecoides, Algal Research, 22 (2017) 122-126.
[20] S. Wang, Y. Wu, X. Wang, Heterotrophic cultivation of
Chlorella pyrenoidosa using sucrose as the sole carbon source by co-culture with
Rhodotorula glutinis, Bioresource Technology, 220 (2016) 615-620.
[21] A. Richmond, Biological principles of mass cultivation, Handbook of microalgal culture: Biotechnology and Applied Phycology, (2004) 125-177.
[22] J. Park, R. Craggs, A. Shilton, Wastewater treatment high rate algal ponds for biofuel production, Bioresource Technology, 102 (2011) 35-42.
[23] W. Zhou, Y. Li, M. Min, B. Hu, H. Zhang, X. Ma, L. Li, Y. Cheng, P. Chen, R. Ruan, Growing wastewater-born microalga
Auxenochlorella protothecoides UMN280 on concentrated municipal wastewater for simultaneous nutrient removal and energy feedstock production, Applied Energy, 98 (2012) 433-440.
[24] J. O’Grady, J.A. Morgan, Heterotrophic growth and lipid production of
Chlorella protothecoides on glycerol, Bioprocess and Biosystems Engineering, 34 (2011) 121-125.
[25] I. Akkerman, M. Janssen, J. Rocha, R.H. Wijffels, Photobiological hydrogen production: photochemical efficiency and bioreactor design, International Journal of Hydrogen Energy, 27 (2002) 1195-1208.
[26] L. Brennan, P. Owende, Biofuels from microalgae. a review of technologies for production, processing, and extractions of biofuels and co-products, Renewable and Sustainable Energy Reviews, 14 (2010) 557-577.
[27] M.A. Borowitzka, Commercial production of microalgae: ponds, tanks, tubes and fermenters, Journal of Biotechnology, 70 (1999) 313-321.
[28] W. Zhou, Y. Li, M. Min, B. Hu, P. Chen, R. Ruan, Local bioprospecting for high-lipid producing microalgal strains to be grown on concentrated municipal wastewater for biofuel production, Bioresource Technology, 102 (2011) 6909-6919.
[29] H.M. Amaro, A.C. Guedes, F.X. Malcata, Advances and perspectives in using microalgae to produce biodiesel, Applied Energy, 88 (2011) 3402-3410.
[30] H. Zheng, Z. Gao, J. Yin, X. Tang, X. Ji, H. Huang, Harvesting of microalgae by flocculation with poly (γ-glutamic acid), Bioresource Technology, 112 (2012) 212-220.
[31] Y. Shen, W. Yuan, Z. Pei, Q. Wu, E. Mao, Microalgae mass production methods, Algae Research, 6 (2009) 1.
[32] J. Zhang, B. Hu, A novel method to harvest microalgae via co-culture of filamentous fungi to form cell pellets, Bioresource Technology, 114 (2012) 529-535.
[33] H. Greenwell, L. Laurens, R. Shields, R. Lovitt, K. Flynn, Placing microalgae on the biofuels priority list: a review of the technological challenges, Journal of the Royal Society Interface, (2009) rsif20090322.
[34] G. Shelef, A. Sukenik, M. Green, Microalgae harvesting and processing: A literature review, Technion Research and Development Foundation Ltd., Haifa (Israel) (1984) 11-48.
[35] S.A. Razzak, M.M. Hossain, R.A. Lucky, A.S. Bassi, H. de Lasa, Integrated CO
2 capture, wastewater treatment and biofuel production by microalgae culturing: a review, Renewable and Sustainable Energy Reviews, 27 (2013) 622-653.
[36] A. Bhatnagar, M. Bhatnagar, S. Chinnasamy, K. Das,
Chlorella minutissima a promising fuel alga for cultivation in municipal wastewaters, Applied Biochemistry and Biotechnology, 161 (2010) 523-536.
[37] Y. Xu, S. Purton, F. Baganz, Chitosan flocculation to aid the harvesting of the microalga
Chlorella sorokiniana, Bioresource Technology, 129 (2013) 296-301.
[38] S.D. Ríos, J. Salvadó, X. Farriol, C. Torras, Antifouling microfiltration strategies to harvest microalgae for biofuel, Bioresource Technology, 119 (2012) 406-418.
[39] J. Edzwald, Algae, bubbles, coagulants, and dissolved air flotation, Water Science and Technology, 27 (1993) 67-81.
[40] E.M. Grima, E.-H. Belarbi, F.A. Fernández, A.R. Medina, Y. Chisti, Recovery of microalgal biomass and metabolites: process options and economics, Biotechnology Advances, 20 (2003) 491-515.
[41] J.J. Milledge, S. Heaven, A review of the harvesting of micro-algae for biofuel production, Reviews in Environmental Science and Biotechnology, 12 (2013) 165-178.
[42] T. Ndikubwimana, X. Zeng, N. He, Z. Xiao, Y. Xie, J.-S. Chang, L. Lin, Y. Lu, Microalgae biomass harvesting by bioflocculation-interpretation by classical DLVO theory, Biochemical Engineering Journal, 101 (2015) 160-167.
[43] C. Wan, M.A. Alam, X.-Q. Zhao, X.-Y. Zhang, S.-L. Guo, S.-H. Ho, J.-S. Chang, F.-W. Bai, Current progress and future prospect of microalgal biomass harvest using various flocculation technologies, Bioresource Technology, 184 (2015) 251-257.
[44] M.A. Alam, D. Vandamme, W. Chun, X. Zhao, I. Foubert, Z. Wang, K. Muylaert, Z. Yuan, Bioflocculation as an innovative harvesting strategy for microalgae, Reviews in Environmental Science and Biotechnology, 15 (2016) 573-583.
[45] D. Mara, Design manual for waste stabilization ponds in India. Leeds: ISBN 0 9519869 1 0, Lagoon Technology International (1997) 17-47.
[46] B. Riaño, B. Molinuevo, M. García-González, Optimization of chitosan flocculation for microalgal-bacterial biomass harvesting via response surface methodology, Ecological Engineering, 38 (2012) 110-113.
[47] S.-H. Ho, C.-Y. Chen, D.-J. Lee, J.-S. Chang, Perspectives on microalgal CO
2-emission mitigation systems: a review, Biotechnology Advances, 29 (2011) 189-198.
[48] P. Talbot, J. De la Noüe, Tertiary treatment of wastewater with Phormidium bohneri (Schmidle) under various light and temperature conditions, Water Research, 27 (1993) 153-159.
[49] T.M. Mata, A.A. Martins, N.S. Caetano, Microalgae for biodiesel production and other applications: a review, Renewable and Sustainable Energy Review, 14 (2010) 217-232.
[50] R. Gutiérrez, I. Ferrer, A. González-Molina, H. Salvadó, J. García, E. Uggetti, Microalgae recycling improves biomass recovery from wastewater treatment high rate algal ponds, Water Research, 106 (2016) 539-549.
[51] J. Park, R. Craggs, A. Shilton, Enhancing biomass energy yield from pilot-scale high rate algal ponds with recycling, Water Research, 47 (2013) 4422-4432.
[52] H.E. Schlichting Jr, Survival of some freshwater algae under extreme environmental conditions, Transactions of the American Microscopical Society, (1974) 610-613.
[53] M. Bayer-Giraldi, E. Jin, P.W. Wilson, Characterization of ice binding proteins from sea ice algae, Plant Cold Acclimation: Methods and Protocols, (2014) 241-253.
[54] A. Ghosh, S. Khanra, M. Mondal, G. Halder, O. Tiwari, S. Saini, T.K. Bhowmick, K. Gayen, Progress toward isolation of strains and genetically engineered strains of microalgae for production of biofuel and other value added chemicals: a review, Energy Conversion and Management, 113 (2016) 104-118.
[55] E.Y. Koh, R.O. Cowie, A.M. Simpson, R. O'toole, K.G. Ryan, The origin of cyanobacteria in Antarctic sea ice: marine or freshwater?, Environmental microbiology reports, 4 (2012) 479-483.
[56] U. Nübel, F. Garcia-Pichel, G. Muyzer, The halotolerance and phylogeny of cyanobacteria with tightly coiled trichomes (
Spirulina turpin) and the description of
Halospirulina tapeticola gen. nov., sp. nov, International Journal of Systematic and Evolutionary Microbiology, 50 (2000) 1265-1277.
[57] R. Massimi, A.E. Kirkwood, Screening microalgae isolated from urban storm-and wastewater systems as feedstock for biofuel, PeerJ, 4 (2016) e2396.
[58] V.T. Duong, Y. Li, E. Nowak, P.M. Schenk, Microalgae isolation and selection for prospective biodiesel production, Energies, 5 (2012) 1835-1849.
[59] E.G. Pringsheim, Pure cultures of Algae: their preparation & maintenance, CUP Archive (1946) 57-77.
[60] J.R. Schramm, Some pure culture methods in the algae, Annals of the Missouri Botanical Garden, 1 (1914) 23-45.
[61] J. Acreman, Algae and cyanobacteria: isolation, culture and long-term maintenance, Journal of Industrial Microbiology & Biotechnology, 13 (1994) 193-194.
[62] M.T. Jahn, K. Schmidt, T. Mock, A novel cost effective and high-throughput isolation and identification method for marine microalgae, Plant methods, 10 (2014) 26.
[63] G.G. Satpati, N. Barman, T. Chakraborty, R. Pal, Unusual habitat of algae, J. Algal Biomass Utln, 2 (2011) 50-52.
[64] L.T. Dinh, Y. Guo, M.S. Mannan, Sustainability evaluation of biodiesel production using multicriteria decision-making, Environmental Progress & Sustainable Energy, 28 (2009) 38-46.
[65] M. Song, H. Pei, W. Hu, S. Zhang, G. Ma, L. Han, Y. Ji, Identification and characterization of a freshwater microalga Scenedesmus SDEC-8 for nutrient removal and biodiesel production, Bioresource Technology, 162 (2014) 129-135.
[66] M.L. Hamilton, S. Powers, J.A. Napier, O. Sayanova, Heterotrophic production of omega-3 long-chain polyunsaturated fatty acids by trophically converted marine diatom phaeodactylum tricornutum, Marine Drugs, 14 (2016) 53.
[67] Z. Tian-Yuan, W. Yin-Hu, Z. Lin-Lan, W. Xiao-Xiong, H. Hong-Ying, Screening heterotrophic microalgal strains by using the Biolog method for biofuel production from organic wastewater, Algal Research, 6 (2014) 175-179.
[68] L. Wang, M. Min, Y. Li, P. Chen, Y. Chen, Y. Liu, Y. Wang, R. Ruan, Cultivation of green algae
Chlorella sp. in different wastewaters from municipal wastewater treatment plant, Applied Biochemistry and Biotechnology, 162 (2010) 1174-1186.
[69] S.B. Ummalyma, R.K. Sukumaran, Cultivation of microalgae in dairy effluent for oil production and removal of organic pollution load, Bioresource Technology, 165 (2014) 295-301.
[70] P. Ramsundar, A. Guldhe, P. Singh, F. Bux, Assessment of municipal wastewaters at various stages of treatment process as potential growth media for
Chlorella sorokiniana under different modes of cultivation, Bioresource Technology, 227 (2017) 82-92.
[71] M. Tale, S. Ghosh, B. Kapadnis, S. Kale, Isolation and characterization of microalgae for biodiesel production from Nisargruna biogas plant effluent, Bioresource Technology, 169 (2014) 328-335.
[72] C. Banerjee, R. Bandopadhyay, P. Shukla, A simple novel agar diffusion method for isolation of indigenous microalgae
Chlamydomonas sp. CRP7 and
Chlorella sp. CB4 from operational swampy top soil, Indian Journal of Microbiology, 52 (2012) 710-712.
[73] O. Garcia, Y. Bashan, M. Puente, Organic carbon supplementation of sterilized municipal wastewater is essential for heterotrophic growth and removing ammonium by the microalgae
Chlorella vulgaris1, Journal of Phycology, 47 (2011) 190-199.
[74] E.W. Becker, Microalgae: Biotechnology and microbiology, NSBN 0521350204, Cambridge University Press, 10 (1994) 9-63.
[75] M. Jimenez-Perez, P. Sanchez-Castillo, O. Romera, D. Fernandez-Moreno, C. Pérez-Martınez, Growth and nutrient removal in free and immobilized planktonic green algae isolated from pig manure, Enzyme and Microbial Technology, 34 (2004) 392-398.
[76] I.T.D. Cabanelas, J. Ruiz, Z. Arbib, F.A. Chinalia, C. Garrido-Pérez, F. Rogalla, I.A. Nascimento, J.A. Perales, Comparing the use of different domestic wastewaters for coupling microalgal production and nutrient removal, Bioresource Technology, 131 (2013) 429-436.
[77] O. Perez-Garcia, F.M. Escalante, L.E. de-Bashan, Y. Bashan, Heterotrophic cultures of microalgae: metabolism and potential products, Water Research, 45 (2011) 11-36.
[78] M. Turner, Microalgae-biotechnology and microbiology: E. W. Becker, Cambridge Studies in Biotechnology (Sir J. Baddiley, N. H. Carey, I. J. Higgins & W. G. Potter, editors), No. 10; Cambridge University Press, Cambridge; 1994; 293 pp.; GBP 40.00, US$ 69.95; ISBN 0-521-35020-4, Journal of Experimental Marine Biology and Ecology, 183 (1994) 300-301.
[79] H. Huppe, D. Turpin, Integration of carbon and nitrogen metabolism in plant and algal cells, Annual Review of Plant Biology, 45 (1994) 577-607.
[80] E.J. Olguín, Dual purpose microalgae–bacteria-based systems that treat wastewater and produce biodiesel and chemical products within a Biorefinery, Biotechnology Advances, 30 (2012) 1031-1046.
[81] Y. Li, Y.-F. Chen, P. Chen, M. Min, W. Zhou, B. Martinez, J. Zhu, R. Ruan, Characterization of a microalga
Chlorella sp. well adapted to highly concentrated municipal wastewater for nutrient removal and biodiesel production, Bioresource Technology, 102 (2011) 5138-5144.
[82] R. Muñoz, C. Köllner, B. Guieysse, B. Mattiasson, Photosynthetically oxygenated salicylate biodegradation in a continuous stirred tank photobioreactor, Biotechnology and Bioengineering, 87 (2004) 797-803.
[83] H. Xu, X. Miao, Q. Wu, High quality biodiesel production from a microalga
Chlorella protothecoides by heterotrophic growth in fermenters, Journal of Biotechnology, 126 (2006) 499-507.
[84] D. Morales-Sánchez, O.A. Martinez-Rodriguez, A. Martinez, Heterotrophic cultivation of microalgae: production of metabolites of commercial interest, Journal of Chemical Technology & Biotechnology, 92 (2017) 925-936.
[85] L. Wang, Y. Li, P. Chen, M. Min, Y. Chen, J. Zhu, R.R. Ruan, Anaerobic digested dairy manure as a nutrient supplement for cultivation of oil-rich green microalgae
Chlorella sp, Bioresource Technology, 101 (2010) 2623-2628.
[86] J. Yang, X. Li, H. Hu, X. Zhang, Y. Yu, Y. Chen, Growth and lipid accumulation properties of a freshwater microalga,
Chlorella ellipsoidea YJ1, in domestic secondary effluents, Applied Energy, 88 (2011) 3295-3299.
[87] Q.-x. Kong, L. Li, B. Martinez, P. Chen, R. Ruan, Culture of microalgae
Chlamydomonas reinhardtii in wastewater for biomass feedstock production, Applied Biochemistry and Biotechnology, 160 (2010) 9-18.
[88] K. Kovar, P. Přibyl, M. Wyss, Microalgae grown under heterotrophic and mixotrophic conditions, Industrial Scale Suspension Culture of Living Cells, (2013) 164-185.
[89] X. Miao, Q. Wu, Biodiesel production from heterotrophic microalgal oil, Bioresource Technology, 97 (2006) 841-846.
[90] Y.-K. Lee, Commercial production of microalgae in the Asia-Pacific rim, Journal of Applied Phycology, 9 (1997) 403-411.
[91] T.J. Lundquist, I.C. Woertz, N. Quinn, J.R. Benemann, A realistic technology and engineering assessment of algae biofuel production, Energy Biosciences Institute, (2010) 1.
[92] X.-M. Shi, H.-J. Liu, X.-W. Zhang, F. Chen, Production of biomass and lutein by
Chlorella protothecoides at various glucose concentrations in heterotrophic cultures, Process Biochemistry, 34 (1999) 341-347.
[93] A. Richmond, Microalgal biotechnology at the turn of the millennium: a personal view, Journal of Applied Phycology, 12 (2000) 441-451.
[94] S. Abinandan, S. Shanthakumar, Challenges and opportunities in application of microalgae (Chlorophyta) for wastewater treatment: a review, Renewable and Sustainable Energy Reviews, 52 (2015) 123-132.
[95] S. Cho, T.T. Luong, D. Lee, Y.-K. Oh, T. Lee, Reuse of effluent water from a municipal wastewater treatment plant in microalgae cultivation for biofuel production, Bioresource Technology, 102 (2011) 8639-8645.
[96] R. Rippka, J. Deruelles, J.B. Waterbury, M. Herdman, R.Y. Stanier, Generic assignments, strain histories and properties of pure cultures of cyanobacteria, Microbiology, 111 (1979) 1-61.
[97] T. Murwanashyaka, L. Shen, J.D. Ndayambaje, Y. Wang, N. He, Y. Lu, Kinetic and transcriptional exploration of
Chlorella sorokiniana in heterotrophic cultivation for nutrients removal from wastewaters, Algal Research, (2016). https://doi.org/10.1016/j.algal.2016.08.002.
[98] T. Mutanda, D. Ramesh, S. Karthikeyan, S. Kumari, A. Anandraj, F. Bux, Bioprospecting for hyper-lipid producing microalgal strains for sustainable biofuel production, Bioresource Technology, 102 (2011) 57-70.
[99] Introduction to freshwater algae. Freshwater algae, John Wiley & Sons, Inc. (2015) 1-42.
[100] R.M. Uclés, Identification of algal strains by PCR amplification and evaluation of their fatty acid profiles for biodiesel production, Louisiana State University, 2012.
[101] M. Alonso, F.C. Lago, J.M. Vieites, M. Espiñeira, Molecular characterization of microalgae used in aquaculture with biotechnology potential, Aquaculture International, 20 (2012) 847-857.
[102] D.E. Hunt, V. Klepac-Ceraj, S.G. Acinas, C. Gautier, S. Bertilsson, M.F. Polz, Evaluation of 23S rRNA PCR primers for use in phylogenetic studies of bacterial diversity, Applied and Environmental Microbiology, 72 (2006) 2221-2225.
[103] W. Ludwig, K. Schleifer, Bacterial phylogeny based on 16S and 23S rRNA sequence analysis, FEMS Microbiology Reviews, 15 (1994) 155-173.
[104] C. Anne, Choosing the right molecular genetic markers for studying biodiversity: from molecular evolution to practical aspects, Genetica, 127 (2006) 101-120.
[105] S. Cho, N. Lee, S. Park, J. Yu, T.T. Luong, Y.-K. Oh, T. Lee, Microalgae cultivation for bioenergy production using wastewaters from a municipal WWTP as nutritional sources, Bioresource Technology, 131 (2013) 515-520.
[106] J.D. Wehr, R.G. Sheath, Freshwater habitats of algae, Freshwater Algae of North America, Academic Press, Burlington (2003) 11-57.
[107] K. Tamura, D. Peterson, N. Peterson, G. Stecher, M. Nei, S. Kumar, MEGA5: molecular evolutionary genetics analysis using maximum likelihood, evolutionary distance, and maximum parsimony methods, Molecular Biology and Evolution, 28 (2011) 2731-2739.
[108] Y. Xie, S.-H. Ho, C.-N.N. Chen, C.-Y. Chen, I.-S. Ng, K.-J. Jing, J.-S. Chang, Y. Lu, Phototrophic cultivation of a thermo-tolerant
Desmodesmus sp. for lutein production: Effects of nitrate concentration, light intensity and fed-batch operation, Bioresource Technology, 144 (2013) 435-444.
[109] M. Axelsson, F. Gentili, A single-step method for rapid extraction of total lipids from green microalgae, PLoS One, 9 (2014) e89643.
[110] D. Manheim, Y. Nelson, Settling and bioflocculation of two species of algae used in wastewater treatment and algae biomass production, Environmental Progress & Sustainable Energy, 32 (2013) 946-954.
[111] M. Sezgin, Variation of sludge volume index with activated sludge characteristics, Water Research, 16 (1982) 83-88.
[112] D.W. Schneider, Process Control as Ecosystem Management: Using the history of sewage treatment plants to analyze ecosystem management practices, Ecosystems, 9 (2006) 1156-1169.
[113] I. Yousuf, Methods for estimation and comparison of activated sludge settleability, 38th Annual WIOA Old Water Industry Operations Conference, Parklands, Gold Coast, 2013.
[114] S.J. Van Vuuren, Easy identification of the most common freshwater algae: A guide for the identification of microscopic algae in South African freshwaters, Resource Quality Services (RQS)2006. ISBN 0-62135471-6.
[115] M.S. Calderon, S.M. Boo, Phylogeny of phyllophoraceae (Rhodophyta, Gigartinales) reveals asterfilopsis gen. nov. from the Southern Hemisphere, Phycologia, 55 (2016) 543-554.
[116] J. Ma, Z. Wang, J. Zhang, T.D. Waite, Z. Wu, Cost-effective
Chlorella biomass production from dilute wastewater using a novel photosynthetic microbial fuel cell (PMFC), Water Research, 108 (2017) 356-364.
[117] F. Lananan, F.H.M. Yunos, N.M. Nasir, N.S.A. Bakar, S.S. Lam, A. Jusoh, Optimization of biomass harvesting of microalgae,
Chlorella sp. utilizing auto-flocculating microalgae,
Ankistrodesmus sp. as bio-flocculant, International Biodeterioration & Biodegradation, 113 (2016) 391-396.
[118] A. Anbalagan, S. Schwede, C.-F. Lindberg, E. Nehrenheim, Influence of hydraulic retention time on indigenous microalgae and activated sludge process, Water Research, 91 (2016) 277-284.
[119] J. Park, R. Craggs, A. Shilton, Recycling algae to improve species control and harvest efficiency from a high rate algal pond, Water Research, 45 (2011) 6637-6649.
[120] H. Wang, W. Zhang, L. Chen, J. Wang, T. Liu, The contamination and control of biological pollutants in mass cultivation of microalgae, Bioresource Technology, 128 (2013) 745-750.
[121] L. Xin, H. Hong-ying, Y. Jia, Lipid accumulation and nutrient removal properties of a newly isolated freshwater microalga,
Scenedesmus sp. LX1, growing in secondary effluent, New Biotechnology, 27 (2010) 59-63.
[122] M.P. Devi, G.V. Subhash, S.V. Mohan, Heterotrophic cultivation of mixed microalgae for lipid accumulation and wastewater treatment during sequential growth and starvation phases: Effect of nutrient supplementation, Renewable Energy, 43 (2012) 276-283.
[123] H.-Y. Ren, B.-F. Liu, C. Ma, L. Zhao, N.-Q. Ren, A new lipid-rich microalga
Scenedesmus sp. strain R-16 isolated using Nile red staining: effects of carbon and nitrogen sources and initial pH on the biomass and lipid production, Biotechnology for Biofuels, 6 (2013).
[124] M. Prathima Devi, G. Venkata Subhash, S. Venkata Mohan, Heterotrophic cultivation of mixed microalgae for lipid accumulation and wastewater treatment during sequential growth and starvation phases: Effect of nutrient supplementation, Renewable Energy, 43 (2012) 276-283.
[125] L. Wang, M. Min, Y. Li, P. Chen, Y. Chen, Y. Liu, Y. Wang, R. Ruan, Cultivation of green algae
Chlorella sp. in different wastewaters from municipal wastewater treatment plant, Applied Biochemistry and Biotechnology, 162 (2010) 1174-1186.
[126] S. Kim, Y. Lee, S.-J. Hwang, Removal of nitrogen and phosphorus by
Chlorella sorokiniana cultured heterotrophically in ammonia and nitrate, International Biodeterioration & Biodegradation, 85 (2013) 511-516.
[127] Q.-H. Shen, J.-W. Jiang, L.-P. Chen, L.-H. Cheng, X.-H. Xu, H.-L. Chen, Effect of carbon source on biomass growth and nutrients removal of
Scenedesmus obliquus for wastewater advanced treatment and lipid production, Bioresource Technology, 190 (2015) 257-263.
[128] K. Matusiak, M. Przytocka-Jusiak, K. Leszczyńska-Gerula, M. Horoch, Studies on the purification of wastewater from the nitrogen fertillizer industry by intensive algal cultures. Removal of nitrogen from the wastewater, Acta Microbiologica Polonica, 25 (1976) 361.
[129] A. Juneja, R.M. Ceballos, G.S. Murthy, Effects of environmental factors and nutrient availability on the biochemical composition of algae for biofuels production: a review, Energies, 6 (2013) 4607-4638.
[130] G.V. Subhash, M. Rohit, M.P. Devi, Y. Swamy, S.V. Mohan, Temperature induced stress influence on biodiesel productivity during mixotrophic microalgae cultivation with wastewater, Bioresource Technology, 169 (2014) 789-793.
[131] S. Chinnasamy, B. Ramakrishnan, A. Bhatnagar, K.C. Das, Biomass production potential of a wastewater alga
Chlorella vulgaris ARC 1 under elevated levels of CO
2 and temperature, International Journal of Molecular Sciences, 10 (2009) 518-532.
[132] L.E. De-Bashan, Y. Bashan, Recent advances in removing phosphorus from wastewater and its future use as fertilizer (1997–2003), Water Research, 38 (2004) 4222-4246.
[133] L. Zhu, Z. Wang, Q. Shu, J. Takala, E. Hiltunen, P. Feng, Z. Yuan, Nutrient removal and biodiesel production by integration of freshwater algae cultivation with piggery wastewater treatment, Water Research, 47 (2013) 4294-4302.
[134] R.J. Craggs, W.H. Adey, B.K. Jessup, W.J. Oswald, A controlled stream mesocosm for tertiary treatment of sewage, Ecological Engineering, 6 (1996) 149-169.
[135] K. Larsdotter, J. la Cour Jansen, G. Dalhammar, Phosphorus removal from wastewater by microalgae in Sweden–a year‐round perspective, Environmental Technology, 31 (2010) 117-123.
[136] G. Roeselers, M. Van Loosdrecht, G. Muyzer, Phototrophic biofilms and their potential applications, Journal of Applied Phycology, 20 (2008) 227-235.
[137] H. Lu, L. Yang, S. Shabbir, Y. Wu, The adsorption process during inorganic phosphorus removal by cultured periphyton, Environmental Science and Pollution Research, 21 (2014) 8782-8791.
[138] S.A. Sañudo-Wilhelmy, A. Tovar-Sanchez, F.-X. Fu, D.G. Capone, E.J. Carpenter, D.A. Hutchins, The impact of surface-adsorbed phosphorus on phytoplankton Redfield stoichiometry, Nature, 432 (2004) 897-901.
[139] T.-Y. Zhang, Y.-H. Wu, L.-L. Zhuang, X.-X. Wang, H.-Y. Hu, Screening heterotrophic microalgal strains by using the Biolog method for biofuel production from organic wastewater, Algal Research, 6 (2014) 175-179.